Chapter 12. Photosynthesis
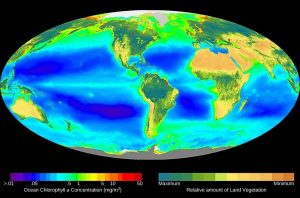
Chapter Outline
- 12.1 Overview of Photosynthesis
- 12.2 The Light-Dependent Reactions of Photosynthesis
- 12.3 Using Light Energy to Make Organic Molecules
Introduction
The processes in all organisms—from bacteria to humans—require energy. To get this energy, many organisms access stored energy by eating, that is, by ingesting other organisms. But where does the stored energy in food originate? All of this energy can be traced back to photosynthesis.
12.1 | Overview of Photosynthesis
Learning Objectives
By the end of this section, you will be able to:
- Explain the relevance of photosynthesis to other living things.
- Describe the main structures involved in photosynthesis.
- Identify the substrates and products of photosynthesis.
- Summarize the process of photosynthesis.
Photosynthesis is essential to all life on earth. It is the only biological process that can capture energy that originates in outer space (sunlight) and convert it into chemical compounds (carbohydrates) that every organism uses to power its metabolism. In brief, the energy of sunlight is used to energize electrons, which are then stored in the covalent bonds of sugar molecules. The energy extracted today by the burning of coal and petroleum products represents sunlight energy captured and stored by photosynthesis almost 200 million years ago.
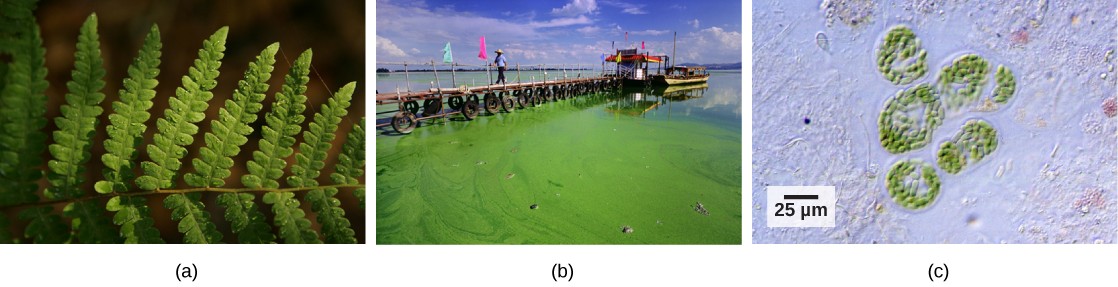
Plants, algae, and a group of bacteria called cyanobacteria are the only organisms capable of performing photosynthesis (Figure 12.2). Because they use light to manufacture their own food, they are called autotrophs (“self-feeders”). Other organisms, such as animals, fungi, and most other bacteria, are termed heterotrophs (“other feeders”), because they must rely on the sugars produced by photosynthetic organisms for their energy needs.
The importance of photosynthesis is not just that it can capture sunlight’s energy. A lizard sunning itself on a cold day can use the sun’s energy to warm up. Photosynthesis is vital because it evolved as a way to store the energy in solar radiation as high-energy electrons in the carbon-carbon bonds of carbohydrate molecules. Those carbohydrates are the energy source that heterotrophs use to power the synthesis of ATP via cellular respiration. Therefore, photosynthesis powers Earth’s ecosystems. When a top predator, such as a wolf, preys on a deer, the wolf is at the end of an energy path that went from nuclear reactions on the surface of the sun, to light, to photosynthesis, to vegetation, to deer, and finally to wolf.
12.1.1 Main Structures and Summary of Photosynthesis
Photosynthesis is a multi-step process that requires sunlight, carbon dioxide, and water as substrates (Figure 12.3). After the process is complete, it releases oxygen and produces glyceraldehyde-3-phosphate (GA3P), simple carbohydrate molecules (which are high in energy) that can subsequently be converted into glucose, sucrose, or any of dozens of other sugar molecules. These sugar molecules contain energy and the energized carbon that all living things need to survive.
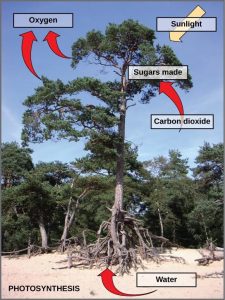
The following is the chemical equation for photosynthesis:
Although the equation looks simple, the many steps that take place during photosynthesis are actually quite complex. Before learning the details of how photoautotrophs use sunlight to synthesize food, it is important to become familiar with the structures involved.
In plants, photosynthesis generally takes place in leaves, which consist of several layers of cells. Photosynthesis occurs in a middle layer called the mesophyll. The gas exchange of carbon dioxide and oxygen occurs through small, regulated openings called stomata (singular: stoma), which also play roles in the regulation of gas exchange and water balance. The stomata are typically located on the underside of the leaf, which helps to minimize water loss. Each stoma is flanked by guard cells that regulate the opening and closing of the stomata by swelling or shrinking in response to osmotic changes (Figure 12.4).
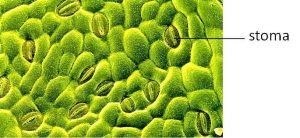
Concept Check
On a hot, dry day, plants close their stomata to conserve water. What impact will this have on photosynthesis?
In all autotrophic eukaryotes, photosynthesis takes place inside an organelle called a chloroplast. For plants, chloroplast-containing cells exist in the mesophyll. Chloroplasts have a double membrane envelope composed of an outer membrane and an inner membrane. Within the chloroplast are stacked, disc-shaped structures called thylakoids. Embedded in the thylakoid membrane is chlorophyll, a pigment (molecule that absorbs light) responsible for the initial interaction between light and plant material, and numerous proteins that make up the electron transport chain. The thylakoid membrane encloses an internal space called the thylakoid lumen. As shown in Figure 12.5, a stack of thylakoids is called a granum, and the liquid-filled space surrounding the granum is called stroma (not to be confused with stoma).
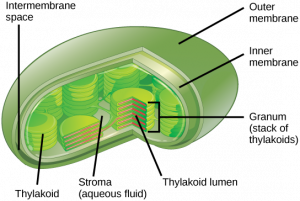
12.1.2 The Two Halves of Photosynthesis
Photosynthesis takes place in two sequential stages: the light-dependent reactions and the light independent-reactions. In the light-dependent reactions, energy from sunlight is absorbed by chlorophyll and that energy is converted into stored chemical energy. In the light-independent reactions, the chemical energy harvested during the light-dependent reactions drives the assembly of sugar molecules from carbon dioxide.
Although the light-independent reactions do not use light directly as a reactant, they require the products of the light-dependent reactions. The light-dependent reactions utilize molecules called energy carriers to temporarily store energy. The energy carriers that move energy from light-dependent reactions to light-independent reactions can be thought of as “full” because they are rich in energy. After the energy is released, the “empty” energy carriers return to the light-dependent reaction to obtain more energy. Figure 12.6 illustrates the components inside the chloroplast where the light-dependent and light-independent reactions take place.
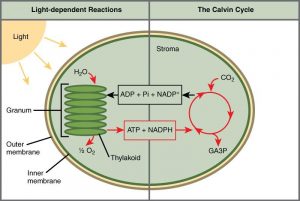
EVERYDAY CONNECTION
Photosynthesis at the Grocery Store
Major grocery stores in the United States are organized into departments, such as dairy, meats, produce, bread, cereals, and so forth. Each aisle (Figure 12.7) contains hundreds, if not thousands, of different products for customers to buy and consume.
Although there is a large variety, each item links back to photosynthesis. Meats and dairy link, because the animals were fed plant-based foods. The breads, cereals, and pastas come largely from starchy grains, which are the seeds of photosynthesis-dependent plants. What about desserts and drinks? All of these products contain sugar—sucrose is a plant product, a disaccharide, a carbohydrate molecule, which is built directly from photosynthesis. Many items are less obviously derived from plants: For instance, paper goods are generally plant products, and many plastics are derived from algae. Virtually every spice and flavoring in the spice aisle was produced by a plant as a leaf, root, bark, flower, fruit, or stem. Ultimately, photosynthesis connects to every meal and every food a person consumes.
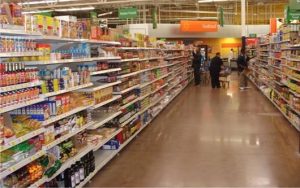
12.2 | The Light-Dependent Reactions of Photosynthesis
Learning Objectives
By the end of this section, you will be able to:
- Explain how plants absorb energy from sunlight.
- Describe short and long wavelengths of light.
- Describe how and where photosynthesis takes place within a plant.
How can light be used to make food? Like all other forms of kinetic energy, light can travel, change form, and be harnessed to do work. In photosynthesis, light energy is converted into chemical energy, which photoautotrophs use to build carbohydrate molecules (Figure 12.8). However, autotrophs only use a few specific components of sunlight.
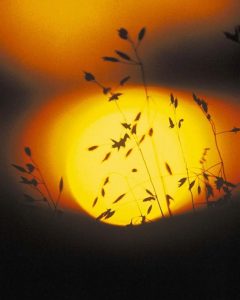
12.2.1 What Is Light Energy?
The sun emits an enormous amount of electromagnetic radiation, or solar energy. Solar energy is composed of tiny, mass-less packets of energy called photons. Humans can see only the tiny fraction of this energy that is capable of stimulating photoreceptor cells in our retinas. This portion is referred to as “visible light.”
The manner in which photons travel is described as waves. Scientists can determine the amount of energy in a particular photon by measuring its wavelength, the distance between consecutive points of a wave. A single wave is measured from two consecutive points, such as from crest to crest or from trough to trough (Figure 12.9).
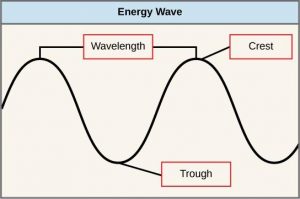
Visible light is only one of many types of electromagnetic radiation emitted from the sun. Scientists describe the various types of radiant energy from the sun on an electromagnetic spectrum. The electromagnetic spectrum is the range of all possible frequencies of radiation, including X-rays, ultraviolet (uv) rays, microwaves, and radio waves (Figure 12.10).
Each type of electromagnetic radiation has photons that travel at a particular wavelength. Since the height of the waves is the same, the wavelength relates to how quickly the photons are vibrating up and down as they travel a given distance. Therefore, the longer the wavelength, the less energy is carried by the photons. Photons traveling in short, tight waves carry the most energy. The higher-energy waves can penetrate tissues and damage cells and DNA, explaining why both X- rays and UV rays can be harmful to living organisms.
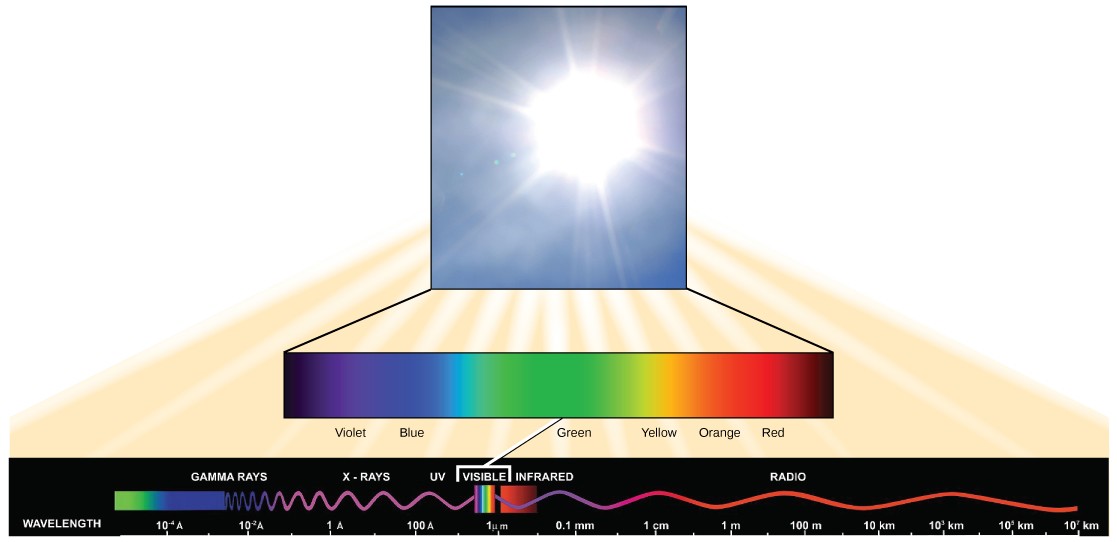
12.2.2 Absorption of Light
Pigments are molecules that absorb a portion of visible light. Interestingly, the portion of visible light that is NOT absorbed by a pigment is reflected to our eyes. Therefore, the color we perceive represents the wavelengths of visible light that are NOT absorbed by a particular pigment. For example, pigments in a red-skinned apple absorb yellow, green and blue light and reflect red light (Figure 12.11).
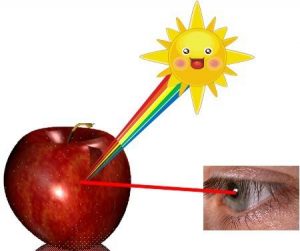
Organic pigments, such as those used for photosynthesis, have a narrow range of energy levels that they can absorb. In fact, they can only absorb the exact amount of energy needed to raise an electron to an excited state. Recall that electrons occupy discrete energy levels in atoms. They can only absorb a photon if it has the exact amount of energy to raise it by one or two energy levels. Photons with different amounts of energy cannot be absorbed (Figure 12.12) Therefore, pigments in our retinas can only “see” (absorb) wavelenghts of 700 nm to 400 nm , which is therefore called visible light. Plant pigment molecules also only absorb light in the wavelength range of 700 nm to 400 nm.
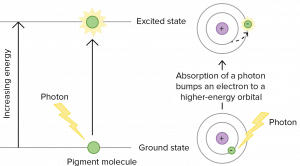
The visible light portion of the electromagnetic spectrum shows a rainbow of colors, with violet and blue having shorter wavelengths, and therefore higher energy. At the red end of the spectrum, the wavelengths are longer and have lower energy (Figure 12.13).
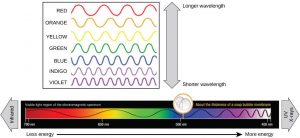
Understanding Pigments
Different kinds of pigments exist, and each has evolved to absorb only certain wavelengths of visible light. Chlorophylls and carotenoids are the two major classes of photosynthetic pigments found in plants and algae. There are five major types of chlorophylls: a, b, c and d and a related molecule found in prokaryotes called bacteriochlorophyll. Chlorophyll a and chlorophyll b are found in higher plant chloroplasts.
With dozens of different forms, carotenoids are a much larger group of pigments. The carotenoids found in fruit—such as the red of tomato (lycopene), the yellow of corn seeds (zeaxanthin), or the orange of an orange peel (β-carotene)—are used to attract seed dispersers. Carotenoids also serve as photosynthetic pigments that reside in the thylakoid membrane, absorb excess energy that could damage the plant, and safely dissipate that energy as heat.
Each type of pigment can be identified by the specific pattern of visible light it absorbs, which can be visualized on an absorption spectrum. Figure 12.14 shows the absorption spectra for chlorophyll a, chlorophyll b, and β-carotene. Notice how each pigment has a distinct set of peaks and troughs, revealing a highly specific pattern of absorption. Chlorophyll a absorbs wavelengths from either end of the visible spectrum (blue and red), but not green. Because green is reflected or transmitted, chlorophyll appears green. Carotenoids absorb in the short-wavelength blue region, and reflect the longer yellow, red, and orange wavelengths.
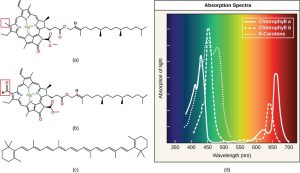
Many photosynthetic organisms have a mixture of pigments, which allows them to absorb energy from a wider range of wavelengths. For example, plants on the rainforest floor must be able to absorb any bit of light that comes through, because the taller trees absorb most of the sunlight and scatter the rest (Figure 12.15).
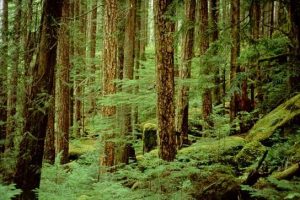
12.2.3 How Light-Dependent Reactions Work
Photosystems
The overall function of light-dependent reactions is to convert solar energy into chemical energy in the form of NADPH and ATP. This chemical energy will fuel the assembly of sugar molecules during the light-independent reactions.
Light energy is converted into chemical energy in photosystems. Photosystems are large multiprotein complexes that consist of hundreds of pigment molecules embedded in a protein matrix (Figure 12.16). Two special pigment molecules are attached to the reaction center, while the rest are part of the antennae complex.
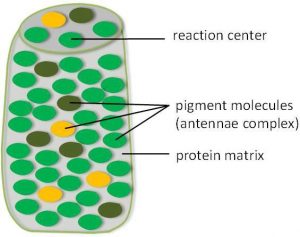
When a photon is absorbed by a pigment molecule in the antennae complex, an electron in that molecule is pushed into an excited state. At this point, the light energy has been captured by a biological molecule but is not yet stored in any useful form. The energy is transferred from chlorophyll to chlorophyll until eventually (after about a millionth of a second), it is delivered to the reaction center. Up to this point, only energy has been transferred between molecules, not electrons.
The reaction center contains a pair of chlorophyll a molecules with a special property. Those two chlorophylls can undergo oxidation upon excitation; they can actually give up an electron. The excited electron is transferred to an electron acceptor and then to an electron transport chain, where its energy is used to make ATP.
The reaction center contains a pair of chlorophyll a molecules with a special property. Those two chlorophylls can undergo oxidation upon excitation; they can actually give up an electron. The excited electron is transferred to an electron acceptor and then to an electron transport chain (ETC), where its energy is used to make ATP in much the same way as in the mitochondria (described below). Since energy from sunlight is used to add phosphate groups to ATP, the process is called photophosphorylation.
Since the reaction center chlorophyll a molecules give up excited electrons, their electrons must be constantly replaced with low energy electrons so that photosynthesis can continue. Two methods have evolved to replace the reaction center electrons. Photosynthetic bacteria use a single photosystem and a process called cyclic photophosphorylation. Plants and algae use two photosystems and a process called non-cyclic photophosphorylation.
Cyclic Photophosphorylation
In the process of cyclic photophosphorylation, bacteria reuse the same electron over and over again. When the reaction center pigment is excited, it transfers an excited electron to an ETC. As the electron is passed down the ETC, its energy is used to set up a proton gradient across folds of the plasma membrane. Protons flow back across the membrane through ATP synthase, turning its rotor, and forcing phosphate groups onto ADP to make ATP. At the bottom of the ETC, most of the electron’s energy has been spent. The low energy electron is returned to the reaction center, where it can be re-excited by solar energy (Figure 12.17a).
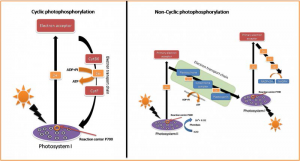
Non-Cyclic Photophosphorylation
In the process of non-cyclic photophosphorylation, plants and algae use two photosystems and the same electrons do not return to be reused (Figure 12.17b). The two types of photosystems, called photosystem II (PSII) and photosystem I (PSI), are found embedded in the thylakoid membrane of chloroplasts. The two complexes differ on the basis of what they oxidize (that is, the source of the low-energy electron supply) and what they reduce (the place to which they deliver their energized electrons) (Figure 12.18).
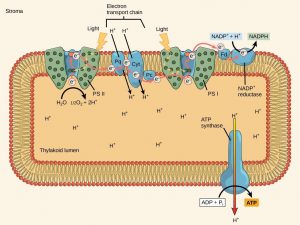
The reaction center of PSII (called P680) delivers its high-energy electrons, one at the time, to an electron acceptor and then through the ETC to PSI. P680’s missing electron is replaced by extracting a low-energy electron from water. Splitting one H2O molecule releases two electrons, two hydrogen atoms, and one atom of oxygen. Splitting two molecules is required to form one molecule of diatomic O2 gas. About 10 percent of the oxygen is used by mitochondria in the leaf to support oxidative phosphorylation. The remainder escapes to the atmosphere where it is used by aerobic organisms to support cellular respiration.
As electrons move through the ETC that resides between PSII and PSI, they lose energy. That energy is used to move hydrogen ions from the stromal side of the membrane to the thylakoid lumen. Those hydrogen ions, plus the ones produced by splitting water, accumulate in the thylakoid lumen and will be used synthesize ATP in a later step. Because the electrons have lost energy prior to their arrival at PSI, they must be re-energized by photons absorbed by the PSI antenna complex. That energy is relayed to the PSI reaction center (called P700). P700 is oxidized and sends a high-energy electron to NADP+ to form NADPH. Thus, PSII captures the energy to create proton gradients to make ATP, and PSI captures the energy to reduce NADP+ into NADPH. The two photosystems work in concert, in part, to guarantee that the production of NADPH will roughly equal the production of ATP. Other mechanisms exist to fine tune that ratio to exactly match the chloroplast’s constantly changing energy needs.
Generating an Energy Carrier: ATP
As in the intermembrane space of the mitochondria during cellular respiration, the buildup of hydrogen ions inside the thylakoid lumen creates a concentration gradient. The exergonic movement of hydrogen ions from high concentration (in the thylakoid lumen) to low concentration (in the stroma) is harnessed to create ATP, just as in the mitochondrial electron transport chain of cellular respiration.
To release the energy stored in the electrochemical gradient, hydrogen ions will rush through any opening, similar to water jetting through a hole in a dam. In the thylakoid, that opening is a passage through a specialized protein channel called ATP synthase. The energy released by the hydrogen ion stream allows ATP synthase to attach a third phosphate group to ADP, which forms a molecule of ATP (Figure 12.18). The flow of hydrogen ions through ATP synthase is called chemiosmosis because the ions move from an area of high to an area of low concentration through a semi-permeable structure.
Concept Check
What is the initial source of electrons for the chloroplast electron transport chain?
- water
- oxygen
- carbon dioxide
- NADPH
12.3 | Using Light Energy to Make Organic Molecules
Learning Objectives
By the end of this section, you will be able to:
- Describe the Calvin cycle.
- Define carbon fixation.
- Explain how photosynthesis works in the energy cycle of all living organisms
After the energy from the sun is converted into chemical energy and temporarily stored in ATP and NADPH molecules, the cell has the fuel needed to build carbohydrate molecules for long-term energy storage. The products of the light-dependent reactions, ATP and NADPH, have lifespans in the range of millionths of seconds, whereas the products of the light-independent reactions (carbohydrates and other forms of reduced carbon) can survive for hundreds of millions of years. The carbohydrate molecules made will have a backbone of carbon atoms. Where does the carbon come from? It comes from carbon dioxide, the gas that is a waste product of respiration in microbes, fungi, plants, and animals.
12.3.1 Light-Independent Reactions: The Calvin Cycle
In plants, carbon dioxide (CO2) enters leaves through stomata and diffuses until it reaches the mesophyll cells. Once in the mesophyll cells, CO2 diffuses into the stroma of the chloroplast—the site of the light-independent reactions. These reactions are sometimes called the Calvin cycle after the scientist who discovered them (Figure 12.19). The light-independent reactions of the Calvin cycle can be organized into three stages: fixation, reduction, and regeneration.
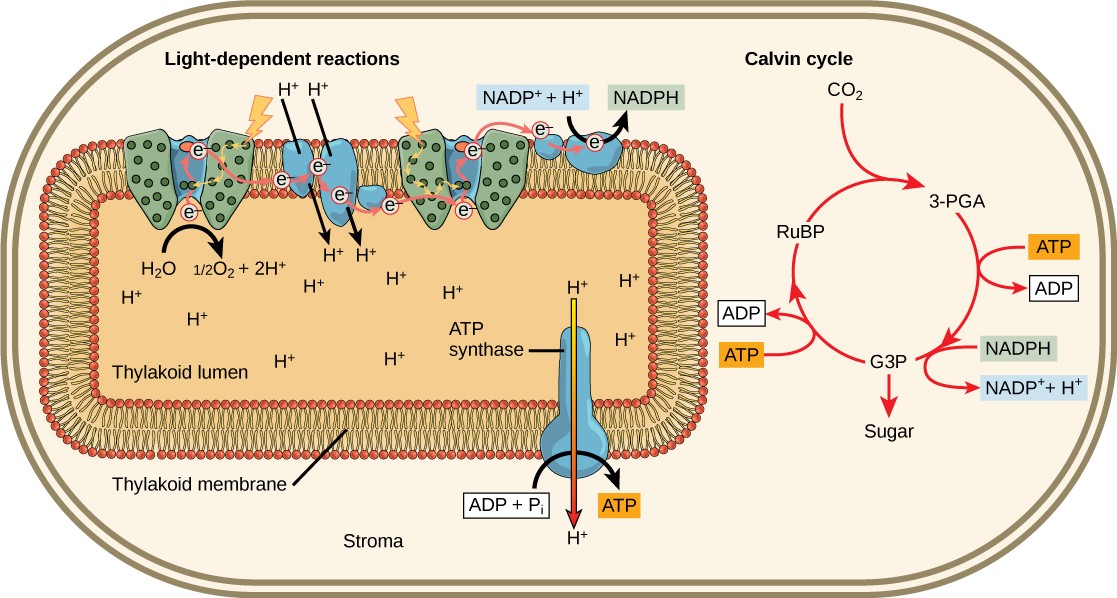
Stage 1: Fixation
In the stroma, in addition to CO2, two other components are present to initiate the light-independent reactions: an enzyme called RuBisCO (ribulose-1,5-bisphosphate carboxylase/oxygenase), and three molecules of RuBP (ribulose bisphosphate). RuBP has five atoms of carbon, flanked by two phosphates (Figure 12.20).
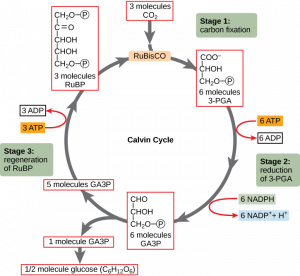
RuBisCO catalyzes a reaction between CO2 and RuBP. For each CO2 molecule that reacts with one RuBP, two molecules of another compound (3-PGA) form. 3-PGA has three carbons and one phosphate. Each turn of the cycle involves only one RuBP and one carbon dioxide and forms two molecules of 3-PGA. Note that the number of carbon atoms remains the same, as the atoms form new bonds. This process is called carbon fixation, because CO2 is “fixed” from an inorganic form into organic molecules.
Stage 2: Reduction
ATP and NADPH are used to convert the six molecules of 3-PGA into six molecules of a chemical called glyceraldehyde 3-phosphate (GA3P). This is a reduction reaction because it involves the gain of electrons by 3-PGA. Six molecules each of ATP and NADPH are used.
Stage 3: Regeneration
Interestingly, at this point, only one of the GA3P molecules leaves the Calvin cycle and is sent to the cytoplasm to contribute to the formation of other compounds needed by the plant. Because the GA3P exported from the chloroplast has three carbon atoms, it takes three “turns” of the Calvin cycle to fix enough net carbon to export one GA3P. Since each turn makes two GA3Ps, three turns make six GA3Ps. The remaining five GA3P molecules remain in the cycle and are used to regenerate RuBP, which enables the system to prepare for more CO2 to be fixed. Three more molecules of ATP are used in these regeneration reactions.
Concept Check
Which of the following statements is true for photosynthesis?
- Oxygen, carbon dioxide, ATP, and NADPH are reactants.
- GA3P and water are products.
- Chlorophyll, water, and carbon dioxide are reactants.
- GA3P and oxygen are products.
- Water, carbon dioxide, ATP, and NADPH are reactants.
- RuBP and oxygen are products.
- In photosynthesis, water and carbon dioxide are reactants. GA3P and oxygen are products.
Photosynthesis
Photosynthesis in desert plants has evolved adaptations that conserve water. In the harsh dry heat, every drop of water must be used to survive. Because stomata must open to allow for the uptake of CO2, water escapes from the leaf during active photosynthesis. Desert plants have evolved processes to conserve water and deal with harsh conditions. A more efficient use of CO2 allows plants to adapt to living with less water. Some plants such as cacti (Figure 12.21) can prepare materials for photosynthesis during the night by a temporary carbon fixation/storage process, because opening the stomata at this time conserves water due to cooler temperatures. In addition, cacti have evolved the ability to carry out low levels of photosynthesis without opening stomata at all, an extreme mechanism to face extremely dry periods.
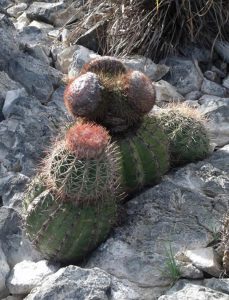
12.3.2 The Energy Cycle
All living things access energy by breaking down carbohydrate molecules. In nature, there is no such thing as waste. Every single atom of matter and energy is conserved, recycling over and over infinitely. Substances change form or move from one type of molecule to another, but their constituent atoms never disappear (Figure 12.22).
CO2 is no more a form of waste than oxygen is wasteful to photosynthesis. Both are byproducts of reactions that move on to other reactions. Photosynthesis absorbs light energy to build carbohydrates in chloroplasts, and aerobic cellular respiration releases energy by using oxygen to metabolize carbohydrates in the cytoplasm and mitochondria. Both processes use electron transport chains to capture the energy necessary to drive other reactions. These two powerhouse processes, photosynthesis and cellular respiration, function in biological, cyclical harmony to allow organisms to access life-sustaining energy that originates millions of miles away in a burning star humans call the sun.
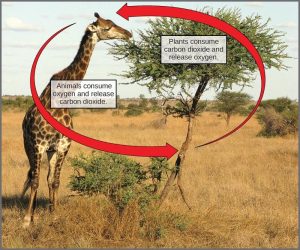