Chapter 2. The Chemical Context of Life
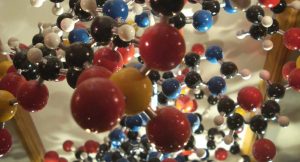
Chapter Outline
- 2.1 Atoms
- 2.2 Chemical Bonding & Intermolecular Forces
- 2.3 Water, Acids & Bases
- 2.4 The Energy of Life
- 2.5 Carbon
Introduction
Elements in various combinations comprise all matter, including living things. Some of the most abundant elements in living organisms include carbon, hydrogen, nitrogen, oxygen, sulfur, and phosphorus. These form the nucleic acids, proteins, carbohydrates, and lipids that are the fundamental components of living matter. Biologists must understand these important building blocks and the unique structures of the atoms that make up molecules, allowing for the formation of cells, tissues, organ systems, and entire organisms.
All biological processes follow the laws of physics and chemistry; so in order to understand how biological systems work, it is important to understand the underlying physics and chemistry. For example, the flow of blood within the circulatory system follows the laws of physics that regulate fluid flow. The breakdown of the large, complex molecules of food into smaller molecules—and the conversion of these to release energy to be stored in adenosine triphosphate (ATP)—is a series of chemical reactions that follow chemical laws. The properties of water and the formation of hydrogen bonds are key to understanding living processes. Recognizing the properties of acids and bases is important, for example, to our understanding of the digestive process. Therefore, the fundamentals of physics and chemistry are important for gaining insight into biological processes.
2.1 | Atoms
Learning Objectives
By the end of this section, you will be able to:
- Define matter and elements.
- Describe the interrelationship between protons, neutrons, and electrons.
- Use atomic number to determine electron configuration.
At its most fundamental level, life is made up of matter: any substance that occupies space and has mass. Elements are unique forms of matter with specific chemical and physical properties that cannot be broken down into smaller substances by ordinary chemical reactions. There are 118 elements, but only 92 occur naturally. The remaining elements are synthesized in laboratories and are unstable.
Each element is designated by its chemical symbol, which is a single capital letter or, when the first letter is already used for another element, a combination of two letters. Some elements follow the English term for the element, such as C for carbon and Ca for calcium. Other elements’ chemical symbols derive from their Latin names; for example, the symbol for sodium is Na, referring to natrium, the Latin word for sodium.
The four most common elements in all living organisms are oxygen (O), carbon (C), hydrogen (H), and nitrogen (N). These elements comprise 96% of living organisms. In the non-living world, elements are found in different proportions, and some elements common to living organisms are relatively rare on the earth as a whole, as shown in Table 2.1. In spite of their differences in abundance, all elements and the chemical reactions between them obey the same chemical and physical laws regardless of whether they are a part of the living or non-living world.
Table 2.1. Percentage of elements in living organisms vs. the non-living world.
Element |
Life (Humans) |
Atmosphere |
Earth’s Crust |
Oxygen (O) |
65% |
21% |
46% |
Carbon (C) |
18% |
trace |
trace |
Hydrogen (H) |
10% |
trace |
0.1% |
Nitrogen (N) |
3% |
78% |
trace |
2.1.1 The Structure of the Atom
An atom is the smallest unit of matter that retains all of the chemical properties of an element. For example, one gold atom has all of the properties of gold, such as that it is a solid metal at room temperature. Gold atoms cannot be broken down into anything smaller while still retaining the properties of gold.
An atom is composed of two regions: the nucleus, which is in the center of the atom and contains protons and neutrons, and the outer region of the atom, which holds its electrons in orbit around the nucleus (Figure 2.2). Atoms contain subatomic particles, the largest of which are protons, electrons, and neutrons.
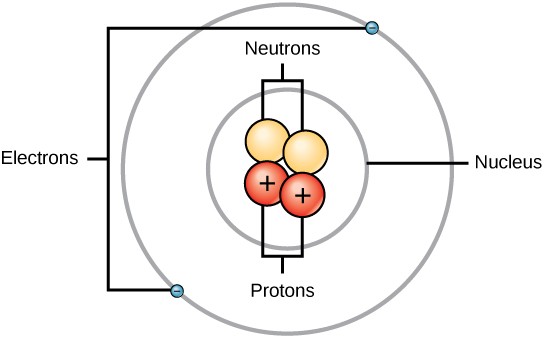
Protons are positively charged, electrons are negatively charged, and neutrons are uncharged (Table 2.2). Each electron has a negative charge equal to the positive charge of a proton. In uncharged, neutral atoms, the number of electrons orbiting the nucleus is equal to the number of protons inside the nucleus. In these atoms, the positive and negative charges cancel each other out, leading to an atom with no net charge.
Protons and neutrons have approximately the same mass, about 1.67 × 10-24 grams. Scientists arbitrarily define this amount of mass as one atomic mass unit (amu) or one Dalton (Da) (Table 2.2). Electrons are much smaller in mass than protons, weighing only 9.11 × 10-28 grams, or about 1/1800 of an atomic mass unit. Hence, they do not contribute much to an element’s overall atomic mass. Therefore, when considering atomic mass, it is customary to ignore the mass of any electrons and calculate the atom’s mass based on the number of protons and neutrons alone.
Accounting for the sizes of protons, neutrons, and electrons, most of the volume of an atom—greater than 99 percent—is, in fact, empty space. With all this empty space, one might ask why so-called solid objects do not just pass through one another. The reason they do not is that the electrons that surround all atoms are negatively charged and negative charges repel each other.
Table 2.2. Properties of subatomic particles.
Charge |
Mass (amu) |
Location |
|
Proton |
+1 |
1 |
nucleus |
Neutron |
0 |
1 |
nucleus |
Electron |
–1 |
0 |
orbitals |
2.1.2 Atomic Number, Mass Number, Isotopes, and Atomic Weight
Atoms of each element contain a characteristic number of protons. The number of protons determines an element’s atomic number and is used to distinguish one element from another.
The number of neutrons in the atoms of a given element is variable. For example, the element carbon (C) has atomic number 6. Therefore, all neutral carbon atoms have 6 protons and 6 electrons. However, some carbon atoms have 6 neutrons, some have 7 neutrons, and some have 8 neutrons. Together, the number of protons plus the number of neutrons determines an atom’s mass number. Note that the small contribution of mass from electrons is disregarded when calculating the mass number.
Isotopes are atoms that have the same number of protons but a different number of neutrons. Carbon atoms that have 6 neutrons have a mass number of 12 amu, and are referred to as Carbon-12 or 12C. Carbon atoms with 7 neutrons have a mass number of 13 amu, and are referred to as Carbon-13 or 13C. Carbon atoms that have 8 neutrons have a mass number of 14 amu, and are referred to as Carbon-14 or 14C. These represent three naturally occurring isotopes of carbon (Figure 2.3).
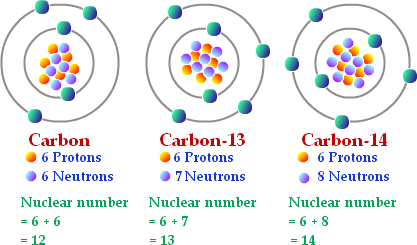
Since an element’s isotopes have different mass numbers, scientists also determine the atomic weight, which is the calculated mean of the mass number for the naturally occurring isotopes of an element on earth. Often, the resulting number is not a whole number. For example, the atomic mass of chlorine (Cl) is 35.45 because chlorine is composed of several isotopes, some (the majority) with atomic mass 35 (17 protons and 18 neutrons) and some with atomic mass 37 (17 protons and 20 neutrons). The atomic mass of carbon is 12.011 because the great majority of carbon on earth is Carbon-12.
Some isotopes may emit neutrons, protons, and electrons in order to become more stable. These are radioactive isotopes, or radioisotopes. Radioactive decay describes the loss of energy and/or mass that occurs when an unstable atom’s nucleus releases radiation. Carbon-14 is an example of a radioisotope (Figure 2.4).

Carbon DatingCarbon-14 (14C) is a naturally occurring radioisotope. In a living organism, the relative amount of 14C is equal to the concentration of 14C in the atmosphere. When an organism dies, the ratio between 14C and 12C will decrease as 14C decays.After approximately 5,730 years, half of the starting concentration of 14C decayed. The time it takes for half of the original concentration of an isotope to decay to its more stable form is called its half-life. The number of half-lives since an object such as bones or wood was alive can be determined by comparing the ratio of the 14C concentration in the object to the amount of 14C detected in the atmosphere. The age of the material can be calculated with accuracy if it is not much older than about 50,000 years (Figure 2.4).
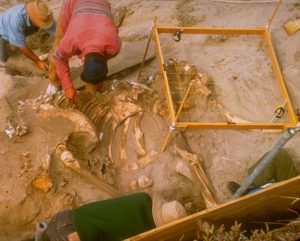
2.1.3 The Periodic Table
The different elements are organized and displayed in the periodic table. Devised by Russian chemist Dmitri Mendeleev (1834–1907) in 1869, the table groups elements that share certain chemical properties. The properties of elements are responsible for their physical state at room temperature: they may be gases, solids, or liquids. Elements also have specific chemical reactivity, the ability to chemically bond with each other.
In the periodic table, shown in Figure 2.5, the elements are organized and displayed according to their atomic number and are arranged in a series of rows and columns based on shared chemical and physical properties. Each square on the table gives the name, chemical symbol, atomic weight, and atomic mass for one element. For example, the first square contains hydrogen, its symbol (H), its atomic number of (1), and its atomic mass (1.01).
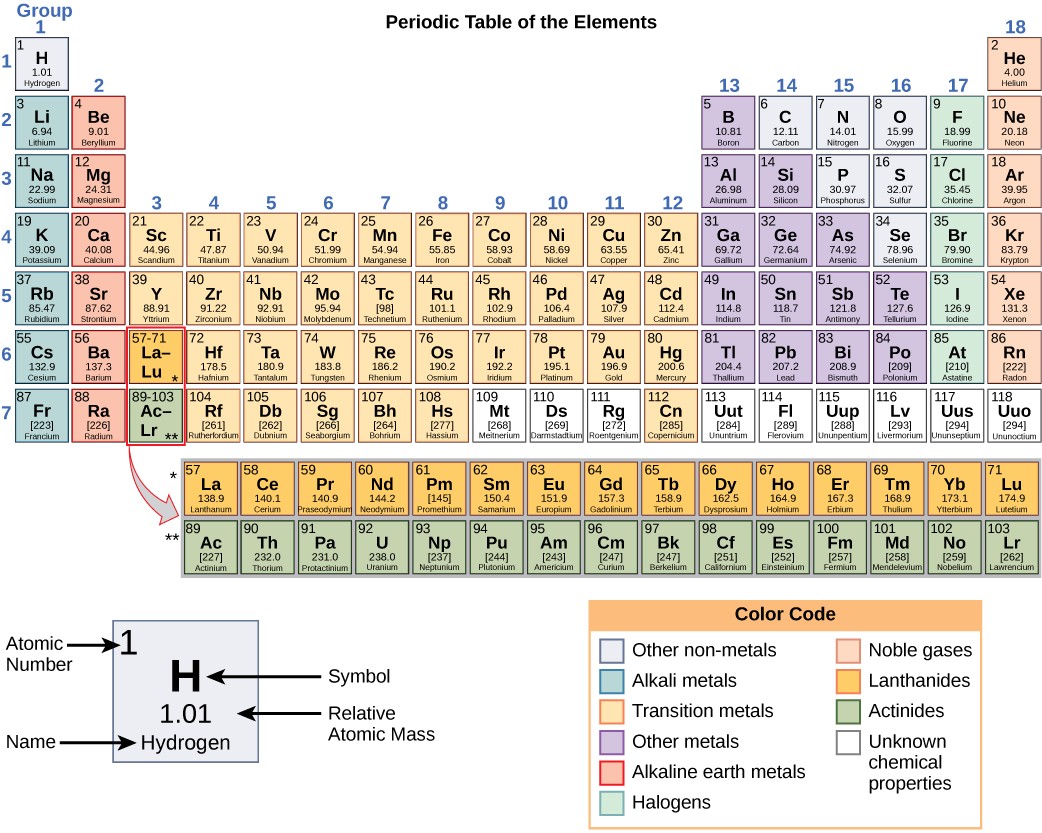
2.1.4 Electron Shells and the Bohr Model
An early model of the atom was developed in 1913 by Danish scientist Niels Bohr (1885–1962). The Bohr model shows the atom with a central nucleus and the electrons in circular electron shells at specific distances from the nucleus. The closer an energy shell is the nucleus, the lower the energy of the electrons that occupy that shell. The first (1n) electron shell can hold two electrons, while the second (2n) and third (3n) shells can hold eight electrons each (Figure 2.6).
Electron Orbitals
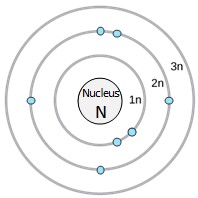
Although useful to explain the reactivity and chemical bonding of certain elements, the Bohr model of the atom does not accurately reflect how electrons are spatially distributed surrounding the nucleus. They do not circle the nucleus like the earth orbits the sun, but are found in three-dimensional electron orbitals. Mathematical equations can predict within a certain level of probability where an electron might be at any given time. The area where an electron is most likely to be found is called its orbital. Each electron orbital can hold only two electrons.
Electrons fill orbitals in a consistent order: they first fill the orbitals closest to the nucleus, then they continue to fill orbitals of increasing energy further from the nucleus. If there are multiple orbitals of equal energy, one electron will be added to each orbital before a second electron is added to any of the orbitals. For example, the second energy level in the nitrogen atom shown here has one electron in each of three orbitals and two electrons in the fourth orbital (Figure 2.6).
The innermost shell has a single orbital, for a maximum of two electrons but the next two electron shells can each have four orbitals, for a maximum of eight electrons. The octet rule states that, with the exception of the innermost shell, atoms are most stable when they have eight electrons in their valence shell, the outermost electron shell. Examples of some neutral atoms and their electron configurations are shown in Figure 2.7.
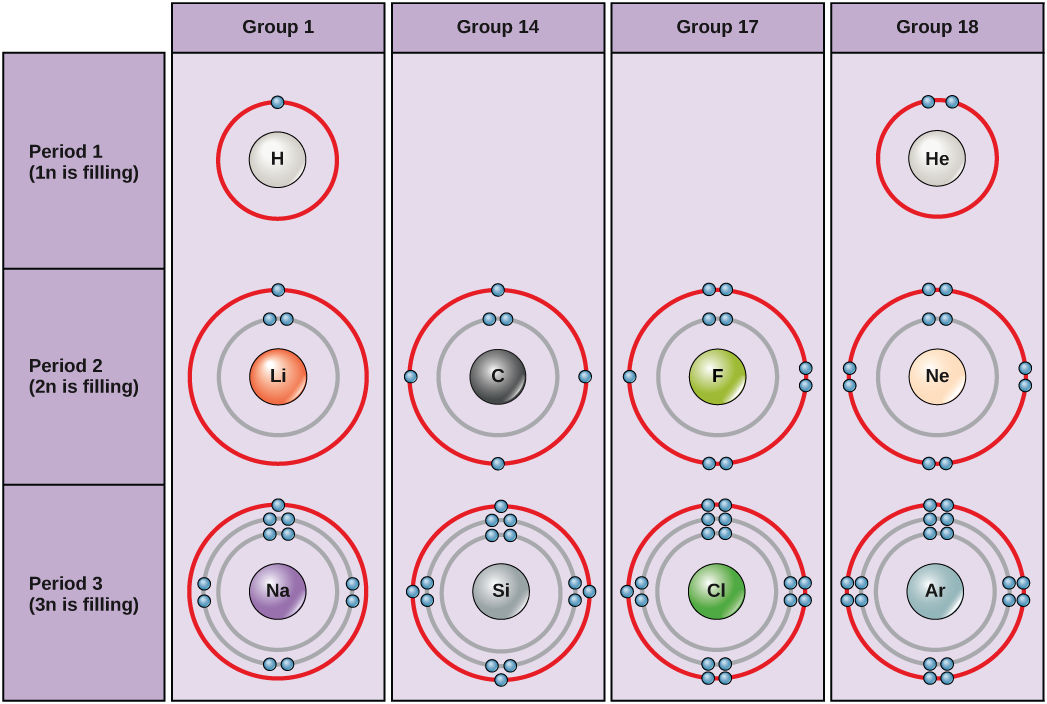
Concept Check
Draw Bohr’s diagrams for oxygen and magnesium atoms.
- How many electrons do oxygen atoms need to gain in order to achieve a stable electron configuration?
- How many electrons do magnesium atoms need to lose to achieve a stable configuration?
The periodic table is arranged in columns and rows based on the number of electrons and where these electrons are located. Note that elements in the far right column of the periodic table (Figure 2.5) all have filled valence shells. These atoms are highly stable, making it unnecessary for them to share electrons with other atoms. They are therefore non-reactive and are called inert gases (or noble gases).
In general, atoms with 4-7 electrons in their valence shell will either gain electrons to become negatively charged ions, or will share electrons with other atoms to form covalently bonded molecules. Atoms with 1-3 electrons in their valence shell will tend to donate their electrons to other atoms until they have a full outer shell. As a result of losing negatively charged electrons, they become positively charged ions.
2.2 | Chemical Bonding & Intermolecular Forces
Learning Objectives
By the end of this section, you will be able to:
- Compare the ways in which electrons can be donated or shared between atoms.
- Explain the ways in which naturally occurring elements combine to create molecules.
- Identify intermolecular forces that hold molecules together.
2.2.1 Chemical Reactions and Molecules
The octet rule drives the chemical behavior of atoms. Atoms will chemically react and bond to each other form molecules, which are simply two or more atoms chemically bonded together. A compound is a type of molecule that contains two or more different types of atoms. In short, atoms form chemical bonds with other atoms, thereby obtaining the electrons they need to attain a stable electron configuration. The familiar water molecule, H2O, consists of two hydrogen atoms and one oxygen atom bonded together (Figure 2.8). Atoms can form molecules by donating, accepting, or sharing electrons to fill their outer shells.
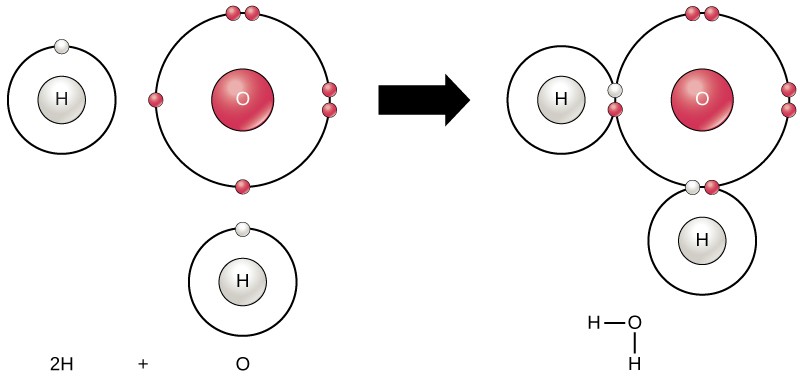
Chemical reactions occur when two or more atoms bond together to form molecules or when bonded atoms are broken apart. The substances used in the beginning of a chemical reaction are called the reactants and the substances found at the end of the reaction are known as the products. An arrow is typically drawn between the reactants and products to indicate the direction of the chemical reaction. Most chemical reactions can go in either direction. For the creation of the water molecule shown above, the chemical equation would be:
2 H2 + O2 → 2 H2O
This is an example of a balanced chemical equation, wherein the number of atoms of each element is the same on each side of the equation.
2.2.2. Ions and Ionic Bonds
Some atoms are more stable when they gain or lose an electrons and form ions. This fills their outermost electron shell and makes them more stable. Because the number of electrons does not equal the number of protons, each ion has a net charge. Cations are positive ions that are formed by losing electrons. Anions are negative ions that are formed by gaining electrons.
Certain ions, such as sodium, potassium, and calcium, are referred to in physiology as electrolytes. These ions are necessary for nerve impulse conduction, muscle contractions and water balance. Many sports drinks and dietary supplements provide these ions to replace those lost from the body via sweating during exercise.
Movement of electrons from one atom or molecule to another is referred to as electron transfer or as a redox reaction. As Figure 2.9 illustrates, sodium (Na) only has one electron in its outer electron shell. If sodium loses an electron, it now has 11 protons and only 10 electrons, making it a sodium cation with an overall charge of +1. Chlorine (Cl) has seven electrons in its outer shell. If it gains an electron, it now has 17 protons and 18 electrons, making it a chloride anion with an overall charge of -1. Both ions now satisfy the octet rule and have complete outermost shells.
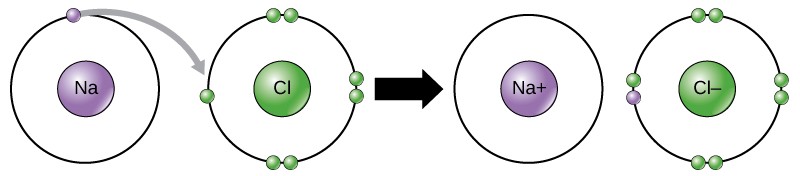
An ionic bond is the electrical attraction that forms between ions with opposite charges. For example, positively charged sodium ions and negatively charged chloride ions bond together to make crystals of sodium chloride, or table salt, creating a crystalline molecule with zero net charge.
2.2.3 Covalent Bonds
Another way the octet rule can be satisfied is by the sharing of electrons between atoms to form covalent bonds. One, two, or three pairs of electrons may be shared, making single, double, and triple bonds, respectively.
The formation of water molecules provides an example of covalent bonding (Figure 2.8). To completely fill the outer shell of oxygen, which has six electrons in its outer shell, two electrons (one from each hydrogen atom) are needed. The electrons are shared between the two elements to fill the outer shell of each, making both elements more stable.
Polar Covalent Bonds
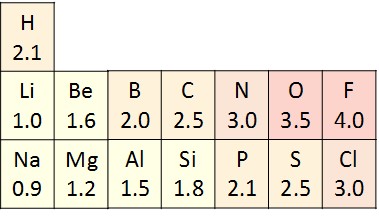
Although atoms share electrons in covalent bonds, they do not always share the electrons equally. Atoms have different electronegativities, or attraction for electrons (Figure 2.10). When a covalent bond is formed between two atoms with different electronegativities, the shared electrons will spend more time around the nucleus of the atom with the higher electronegativity and less time around the nucleus with lower electronegativity. Since electrons are negatively charged, the atom that gets more time with the electron acquires a slightly negative charge (δ–). The atom with lower electronegativity gets less time with the electron and acquires a slightly positive charge (δ+).
The type of covalent bond that forms between two atoms with different electronegativities is called a polar covalent bond. Molecules with polar covalent bonds are called polar molecules, due to the separation of charges across the molecule. For example, water is a polar molecule, since oxygen has an electronegativity of 3.5 and hydrogen has an electronegativity of 2.1. The oxygen atom in a water molecule attracts the shared electrons more and acquires a partial negative charge, while the hydrogen atoms attract the shared electrons less and acquire a partial positive charge (Figure 2.11). Many of the important properties of water result from its polarity.
Nonpolar Covalent Bonds
Nonpolar covalent bonds form between two atoms of the same element or between different elements that share electrons equally. For example, molecular oxygen (O2) is nonpolar because the electrons will be equally distributed between the two oxygen atoms.
Another example of a nonpolar covalent bond is methane (CH4), also shown in Figure 2.11. Carbon and hydrogen have similar electronegativity values. Therefore, these elements share electrons equally, creating a nonpolar covalent molecule. Some molecules are nonpolar due to symmetry, as seen in the carbon dioxide molecule in Figure 2.11.
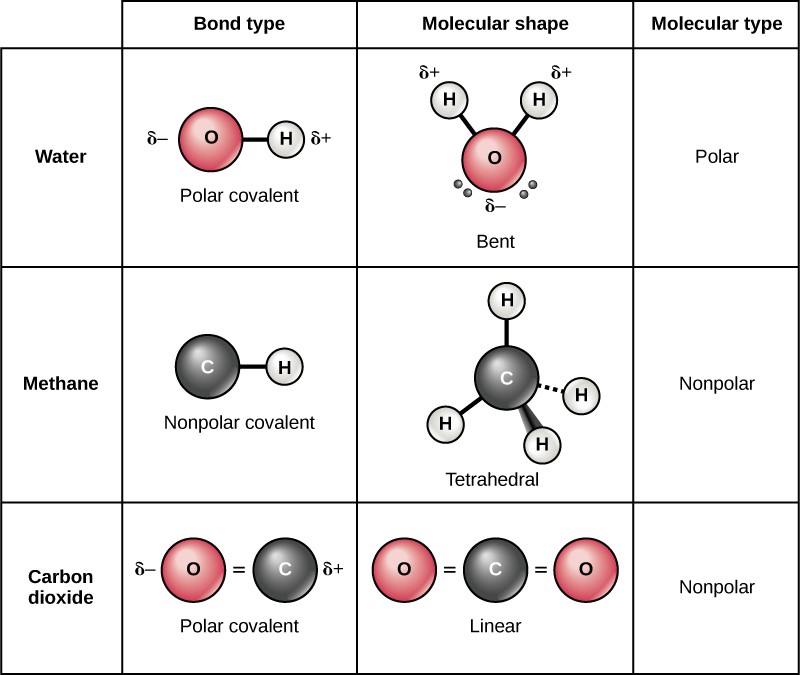
2.2.4 Hydrogen Bonds and Van Der Waals Interactions
As described above, covalent and ionic bonds occur between atoms to form molecules. Other types of interactions occur between molecules and are therefore called intermolecular forces. Two examples of weak attractions that occur frequently between molecules are hydrogen bonds and van der Waals interactions. Without these two types of attractions, life as we know it would not exist.
Hydrogen bonds are weak interactions between two polar molecules or between partially charged parts of molecules. The δ+ of the hydrogen from one molecule is attracted to the δ– charge on the more electronegative atoms (usually oxygen or nitrogen) of another molecule. Hydrogen bonds can also occur between different parts of the same molecule. Individual hydrogen bonds are weak and easily broken; however, they occur in very large numbers in water and in organic polymers, forming very strong cumulative interactions. Hydrogen bonds between water molecules provide many of the critical, life-sustaining properties of water and also stabilize the structures of proteins and DNA.
Like hydrogen bonds, van der Waals interactions are weak attractions or interactions between molecules. Van der Waals attractions can occur between any two or more molecules and are dependent on slight fluctuations of the electron densities, around an atom. For these attractions to happen, the molecules need to be very close to one another. Although weaker than hydrogen bonds, van der Waals interactions are also additive and can be quite strong in great numbers.
Pharmaceutical Chemist
Pharmaceutical chemists are responsible for developing new drugs and for trying to determine the mode of action of drugs. Drugs can be found in nature or can be synthesized in the laboratory. In many cases, potential drugs found in nature are changed chemically in the laboratory to make them safer and more effective.
After the initial discovery or synthesis of a drug, the chemist develops the drug, perhaps by chemically altering it, testing to see if it is toxic, and designing methods for large-scale production. Next, the process of getting the drug approved for human use by the Food and Drug Administration (FDA) begins. This involves a series of large-scale experiments using human subjects to make sure the drug is safe and effective. Approval often takes several years and requires the participation of physicians and chemists.
An example of a drug that was originally discovered in a living organism is Paclitaxel (Taxol), an anti-cancer drug used to treat breast cancer. This drug was discovered in the bark of the pacific yew tree. Another example is aspirin, which was originally isolated from willow tree bark. Both of these drugs are now produced synthetically. Finding drugs often means testing hundreds of samples of plants, fungi, and other forms of life to see if any biologically active compounds are found within them. Sometimes, traditional medicine can give modern medicine clues to where an active compound can be found. For example, the use of willow bark to make medicine has been known for thousands of years, dating back to ancient Egypt. It was not until the late 1800s, however, that the aspirin molecule, known as acetylsalicylic acid, was purified and marketed for human use.
Occasionally, drugs developed for one use are found to have unforeseen effects that allow these drugs to be used in other, unrelated ways. For example, the drug minoxidil (Rogaine) was originally developed to treat high blood pressure. When tested on humans, it was noticed that individuals taking the drug would grow new hair. Eventually the drug was marketed to men and women with baldness to restore lost hair.
The career of the pharmaceutical chemist may involve detective work, experimentation, and drug development, all with the goal of making human beings healthier.
2.3 | Water, Acids & Bases
Learning Objectives
By the end of this section, you will be able to:
- Describe the properties of water that are critical to maintaining life.
- Explain why water is an excellent solvent.
- Provide examples of water’s cohesive and adhesive properties.
- Discuss the role of acids, bases, and buffers in homeostasis.
Why do scientists spend time looking for water on other planets? It is because water is essential to life as we know it. Water is one of the more abundant molecules and the one most critical to life on Earth. Approximately 60–70 percent of the human body is made up of water. Without it, life as we know it simply would not exist.
The polarity of the water molecule and its resulting hydrogen bonding make water a unique substance with special properties that are intimately tied to the processes of life. Life originally evolved in a watery environment, and most of an organism’s cellular chemistry and metabolism occur inside the watery contents of the cell’s cytoplasm. Special properties of water include its high heat capacity and heat of vaporization, its ability to dissolve polar molecules, its cohesive and adhesive properties, and its dissociation into ions that leads to the generation of pH. Understanding these characteristics of water helps to elucidate its importance in maintaining life.
2.3.1 The Properties of Water
The Polarity of Water
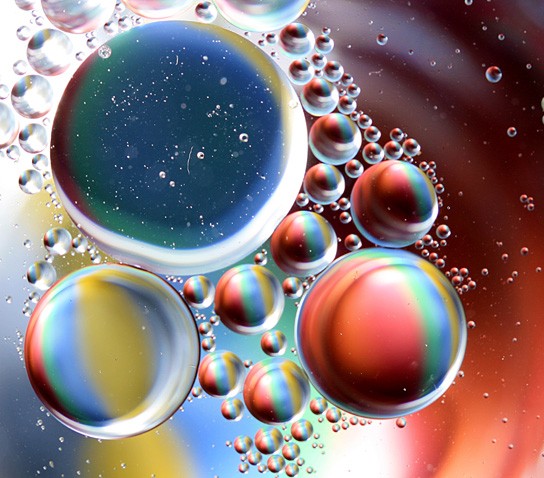
One of water’s important properties is that it is composed of polar molecules. While there is no net charge to a water molecule, the slight positive charges on the hydrogen atoms and the slight negative charges on the oxygen atoms contribute to water’s properties of attraction.
As a result of their polarity, water molecules form hydrogen bonds with each other. Water also attracts, or is attracted to, other polar molecules and ions. A polar substance that interacts readily with or dissolves in water is referred to as hydrophilic (hydro- = “water”; -philic = “loving”). In contrast, non-polar molecules, such as oils and fats, do not interact well with water, and separate from it rather than dissolving in it (Figure 2.12). These nonpolar compounds are called hydrophobic (hydro- = “water”; -phobic = “fearing”).
Water’s States: Gas, Liquid, and Solid
Its many hydrogen bonds cause water to have some unique chemical characteristics compared to other liquids. Since living things have a high water content, understanding these chemical features is key to understanding life. In liquid water, hydrogen bonds constantly form and break as the water molecules slide past each other. The bonds break due to the motion (kinetic energy) of the water molecules due to the heat contained in the system. As water is boiled, the higher kinetic energy of the water molecules causes the hydrogen bonds to break completely and allows water molecules to escape into the air as gas (steam or water vapor).
On the other hand, when water freezes, the water molecules form a crystalline structure that makes ice less dense than liquid water. Water is less dense as a solid because as water freezes the water molecules are pushed farther apart by the hydrogen bonds. In most other substances, molecules pack more tightly as they freeze, making the solid more dense than the liquid. The lower density of ice causes it to float on liquid water (Figure 2.13). In lakes and ponds, ice will form on the surface of the water creating an insulating barrier that protects the living organisms in the pond from freezing. Without this layer of insulating ice, organisms living in the pond would freeze in the solid block of ice and could not survive.
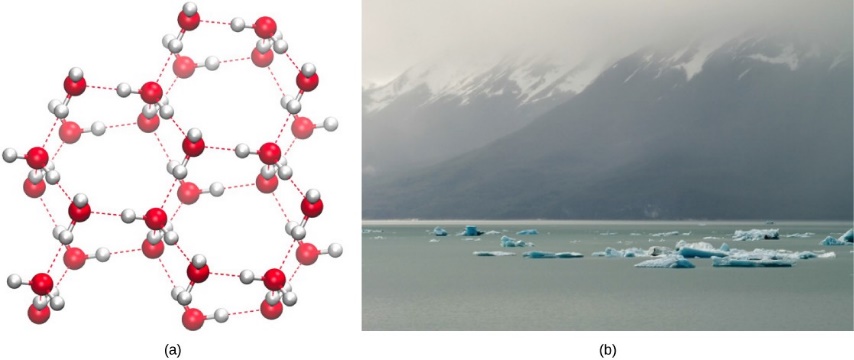
Water’s High Heat Capacity
Water has the highest specific heat capacity of any liquid. Specific heat is defined as the amount of heat one gram of a substance must absorb or lose to change its temperature by one degree Celsius. Because of all of the hydrogen bonds between water molecules, it takes water a long time to heat and long time to cool. In fact, the specific heat capacity of water is about five times more than that of sand. This explains why the land cools faster than the sea. Due to its high heat capacity, water is used by warm blooded animals to help maintain an even temperature.
Water’s Heat of Vaporization
Water also has a high heat of vaporization, the amount of energy required to change one gram of a liquid substance to a gas. A considerable amount of heat energy is required to accomplish this change in water. As liquid water heats up, hydrogen bonding makes it difficult to separate the liquid water molecules from each other, in order for it to enter its gaseous phase (steam). As a result, water requires much more heat to boil than most other liquids. Eventually, as water reaches its boiling point, the heat is able to break the hydrogen bonds between the water molecules, and the kinetic energy (motion) between the water molecules allows them to escape from the liquid as a gas.
Even when below its boiling point, individual water molecules acquire enough energy from other water molecules that some can escape, in a process known as evaporation. Since the evaporation of water requires heat energy, it cools the environment where the evaporation is taking place. In many living organisms, including humans, the evaporation of sweat allows the organism to cool so that homeostasis of body temperature can be maintained.
Water’s Solvent Properties
Since water is a polar molecule with slightly positive and slightly negative charges, ions and polar molecules can readily dissolve in it. Therefore, water is referred to as a solvent, a substance capable of dissolving other molecules. The substance that is dissolved in a liquid is called a solute. The mixture of a solute dissolved in a solvent is called a solution. If the solvent is water, the solution is called an aqueous solution.
Due to their charge, polar molecules and ions form hydrogen bonds with water and become surrounded by water molecules. The resulting sphere of hydration, or hydration shell, serves to keep the particles separated in the water. When ionic compounds are added to water, the individual ions react with the water molecules and their ionic bonds are disrupted in the process of dissociation. For example, when crystals of table salt (NaCl, or sodium chloride) are added to water, the molecules of NaCl dissociate into Na+ and Cl– ions, and spheres of hydration form around the ions. The positively charged sodium ion is surrounded by the partially negative charge of the water molecule’s oxygen. The negatively charged chloride ion is surrounded by the partially positive charge of the hydrogen on the water molecule (Figure 2.14).
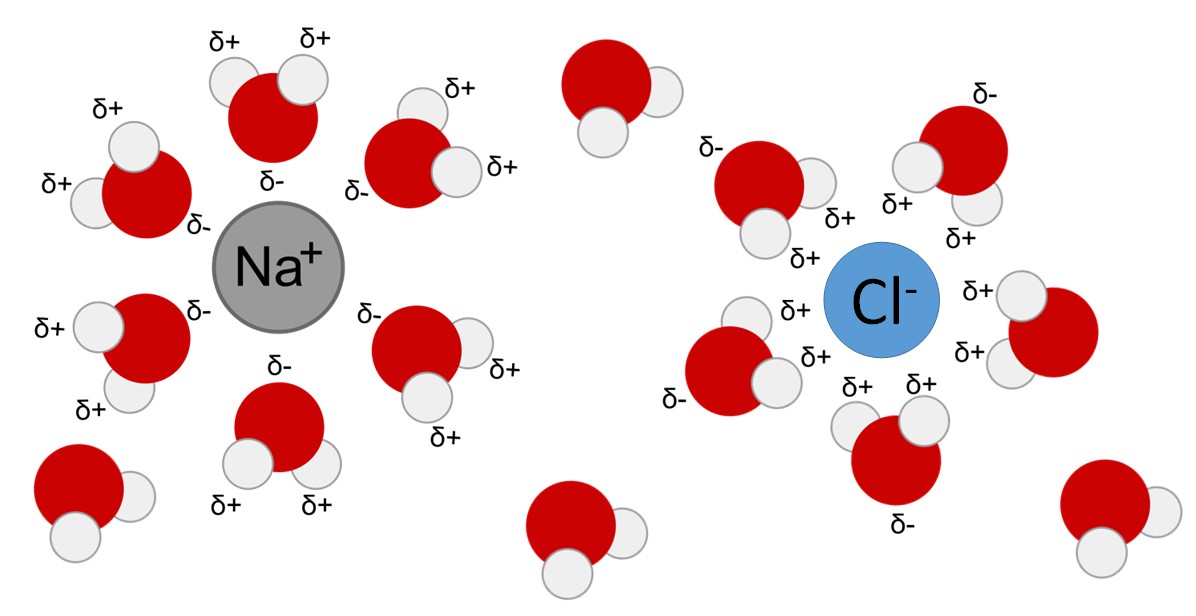
Water’s Cohesive and Adhesive Properties
Have you ever filled a glass of water to the very top and then slowly added a few more drops? Before it overflows, the water forms a dome-like shape above the rim of the glass. This water can stay above the glass because of the property of cohesion. In cohesion, water molecules are attracted to each other, keeping the molecules together at the water-air interface.
Cohesion allows for the development of surface tension, the capacity of a substance to withstand being ruptured when placed under tension or stress. This is also why water forms droplets when placed on a dry surface rather than being flattened out by gravity. It is even possible to “float” a needle on top of a glass of water or for a water strider to stay afloat on the surface layer of water (Figure 2.15).
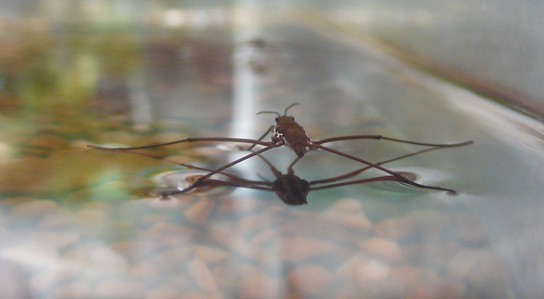
Hydrogen bonding also leads to water’s property of adhesion, or the attraction of water molecules to other molecules. Adhesion is observed when water “climbs” up the tube placed in a glass of water: notice that the water appears to be higher on the sides of the tube than in the middle. This is because the water molecules are attracted to the charged glass walls of the capillary and therefore adhere to it. This type of adhesion is called capillary action (Figure 2.16).
Cohesion and adhesion are important for the transport of water from the roots to the leaves in plants. As water molecules are evaporated from the surface of leaves, they tend to stay connected to water molecules below them, creating a “pull” up the water column. Ultimately, water is pulled into the roots, allowing the plant to receive the dissolved minerals they require from the soil.
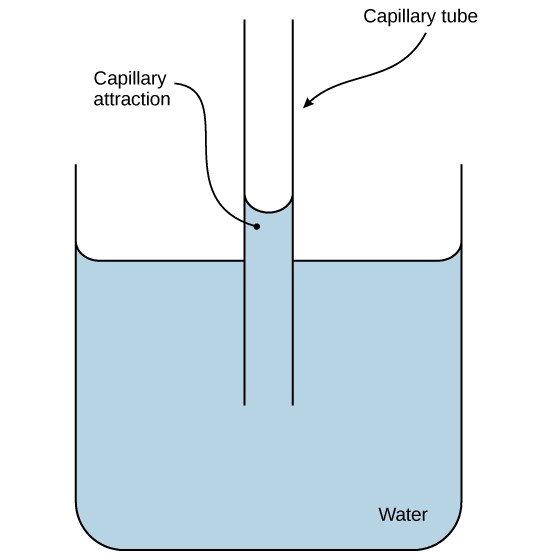
2.3.2 Acids and Bases
pH is a measure of the concentration of hydrogen ions [H+]in a solution. Hydrogen ions are spontaneously generated in pure water by the dissociation (ionization) of a small percentage of water molecules into equal numbers of hydrogen ions (H+) and hydroxide ions (OH–) ions. While the hydroxide ions are kept in solution by hydrogen bonding with other water molecules, the hydrogen ions are immediately attracted to water molecules, forming hydronium ions (H30+). Still, by convention, scientists refer to hydrogen ions and their concentration as if they were free in this state in liquid water.
H2O ↔ H++OH–
H+ +H2O ↔H3O+
The concentration of hydrogen ions dissociating from pure water is 1 × 10-7 moles H+ ions per liter of water. One mole of a substance is equal to 6.02 x 1023 particles of the substance. pH is calculated as the negative of the base 10 logarithm of the H+ concentration. The log10 of 1 × 10-7 is -7.0, and the negative of this number yields a pH of 7.0, which is also known as neutral pH. The pH inside cells (6.8) and the pH of human blood (7.4) are both very close to neutral. Extremes in pH in either direction are usually considered inhospitable to life.
An acid is a substance that increases the H+ concentration in a solution, usually by having one of its hydrogen atoms dissociate. A base provides either OH– or other negatively charged ions that combine with hydrogen ions, reducing their concentration in the solution and thereby raising the pH. In cases where the base releases hydroxide ions, these ions bind to free hydrogen ions, generating new water molecules.
The stronger the acid, the more readily it donates H+. For example, hydrochloric acid (HCl) completely dissociates into hydrogen and chloride ions and is highly acidic, whereas the acids in tomato juice or vinegar do not completely dissociate and are considered weak acids. Conversely, strong bases are those substances that readily donate OH– or take up hydrogen ions. Sodium hydroxide (NaOH) and many household cleaners are highly alkaline and give up OH– rapidly when placed in water, the
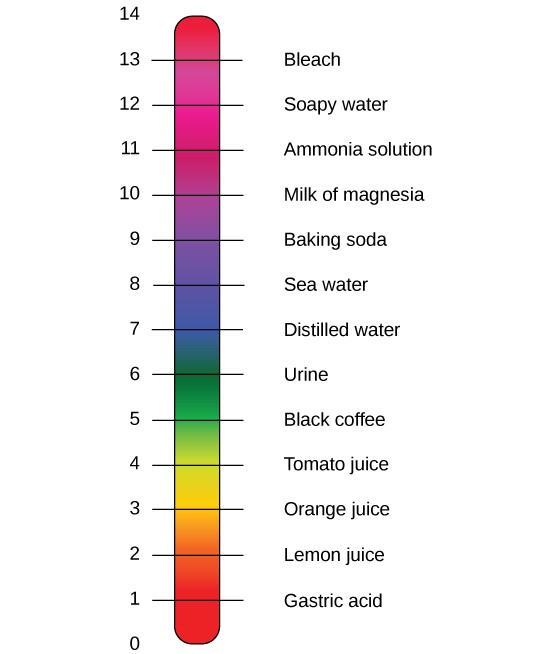
reby raising the pH. An example of a weak basic solution is seawater, which has a pH near 8.0, close enough to neutral pH that marine organisms adapted to this saline environment are able to thrive in it.
The pH scale ranges from 0 to 14 (Figure 2.17). Anything below 7.0 is acidic, and anything above 7.0 is basic, or alkaline. Since the pH scale is a negative logarithmic scale, a ten-fold change in [H+] results in a change of one in pH in the opposite direction. For example, increasing the [H+] from 1 x 10-4 to 1 x 10-3 decreases the pH from pH 4 to pH 3.
So how can organisms that require a near-neutral pH ingest acidic and basic substances (a human drinking orange juice, for example) and survive? Buffers are the key. Buffers readily absorb excess H+ or OH–, keeping the pH of the body in homeostasis. For example, the buffer maintaining the pH of human blood is a mixture of carbonic acid (H2CO3), bicarbonate ion (HCO–), and carbon dioxide (CO2). When bicarbonate ions combine with free hydrogen ions and become carbonic acid, hydrogen ions are removed, moderating pH increases. Excess carbonic acid can be converted to carbon dioxide gas and exhaled through the lungs. This prevents too many free hydrogen ions from building up in the blood and dangerously reducing the blood’s pH. Conversely, if too much OH– is introduced into the system, carbonic acid will combine with it to create bicarbonate, lowering the pH. Without this buffer system, the body’s pH would fluctuate enough to put survival in jeopardy (Figure 2.18).

Other examples of buffers are antacids used to combat excess stomach acid. Many of these over-the-counter medications work in the same way as blood buffers, usually with at least one ion capable of absorbing hydrogen and moderating pH, bringing relief to those that suffer “heartburn” after eating.
2.4 | Energy and Life
Learning Objectives
By the end of this section, you will be able to:
- Explain the concept of metabolism.
- Explain the difference between potential and kinetic energy.
- Explain how the first two laws of thermodynamics relate to living organisms.
2.4.1 Metabolism
Virtually every task performed by living organisms requires energy. In fact, the living cells of every organism constantly use energy. Just as energy is required to both build and demolish a building, energy is required for both the synthesis and breakdown of molecules. Other cellular process that require energy include transport of signaling molecules, such as hormones and neurotransmitters; ingesting and breaking down pathogens, such as bacteria and viruses; importing nutrients and exporting waste; and many others.
The cellular processes listed above require a steady supply of energy. From where, and in what form, does this energy come? How do living cells obtain energy, and how do they use it? This section will discuss different forms of energy and the physical laws that govern energy transfer.
Cellular processes such as building and breaking down complex molecules occur through series of chemical reactions. All of the chemical reactions that take place inside cells, including those that use energy and those that release energy, are the cell’s metabolism. Chemical reactions that require energy to synthesize complex molecules from simpler ones are called anabolic reactions, and chemical reactions that release energy as complex molecules are broken down are called catabolic reactions.
2.4.2. Potential vs. Kinetic Energy
Energy is defined as the capacity to do work. When an object is in motion, there is energy associated with that object because moving objects are capable of enacting a change, or doing work. Think of a wrecking ball. Even a slow-moving wrecking ball can do a great deal of damage to other objects. However, a wrecking ball that is not in motion is incapable of performing work. Energy associated with objects in motion is called kinetic energy. A speeding bullet, a walking person, the rapid movement of molecules in the air, and electromagnetic radiation all have kinetic energy.
Now what if that same motionless wrecking ball is lifted two stories above a car with a crane? If the suspended wrecking ball is unmoving, is there energy associated with it? The answer is yes. The suspended wrecking ball has energy that results from the fact that there is the potential for the wrecking ball to do work. This type of energy is called potential energy. Another example of potential energy is the energy of water held behind a dam (Figure 2.19).
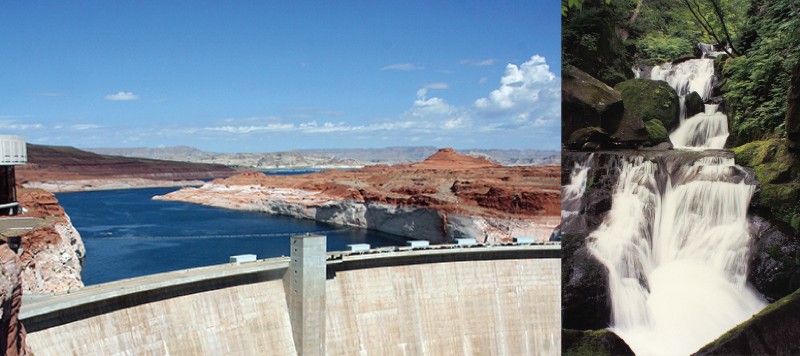
Potential energy is not only associated with the location of matter (such as a wrecking ball being held up), but also with the structure of matter. A spring on the ground has potential energy if it is compressed; so does a rubber band that is pulled taut.
Living cells rely heavily on structural potential energy. On a chemical level, the bonds that hold the atoms of molecules together have potential energy. The fact that energy can be released by the breakdown of certain chemical bonds implies that those bonds have potential energy. In fact, there is potential energy stored within the bonds of all the food molecules we eat, because these bonds can release energy when broken. This type of potential energy is called chemical energy (Figure 2.20). Chemical energy provides cells with energy by breaking the molecular bonds within fuel molecules.
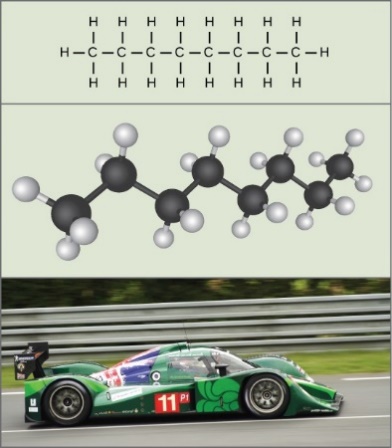
2.4.3 The Laws of Thermodynamics
Thermodynamics refers to the study of energy and energy transfer. The laws of thermodynamics govern the transfer of energy in and among all systems in the universe. The first and second laws of thermodynamics are relevant to biological systems and how they convert and exchange energy with their surroundings.
The First Law of Thermodynamics
The first law of thermodynamics states that the total amount of energy in the universe is constant. In other words, energy cannot be created or destroyed. However, energy may be transferred from one form to another. Transfers and transformations of energy take place around us all the time. Light bulbs transform electrical energy into light energy. Gas stoves transform chemical energy from natural gas into heat energy. Plants convert energy of sunlight into chemical energy stored within organic molecules. Some examples of energy transformations are shown in Figure 2.21.
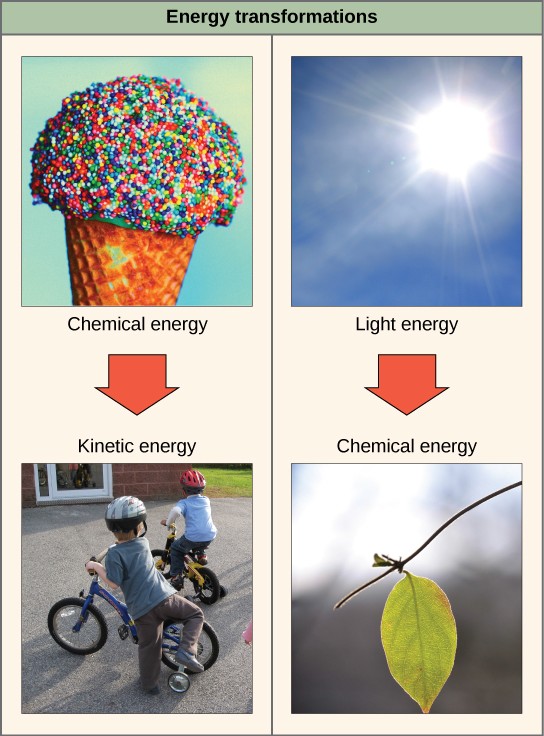
The challenge for all living organisms is to obtain energy from their surroundings in forms that they can transfer or transform into usable energy to do work. Living cells have evolved to meet this challenge very well. Chemical energy stored within organic molecules such as sugars and fats is transformed through a series of cellular chemical reactions into energy within molecules of ATP. Energy in ATP molecules is easily accessible to do work.
The Second Law of Thermodynamics
The second law of thermodynamics states that the disorder, or entropy, in the universe is always increasing. None of the energy transfers and transformations in the universe is completely efficient. In every energy transfer, some amount of energy is lost in a form that is unusable. The more energy that is lost by a system to its surroundings, the less ordered and more random the system is.
In most cases, the energy is lost in the form of heat energy. Thermodynamically, heat energy is the energy transferred from one system to another that is not doing work. For example, when an airplane flies through the air, some of the energy of the flying plane is lost as heat energy due to friction with the surrounding air. This friction actually heats the air by temporarily increasing the speed of air molecules. Likewise, during cellular metabolic reactions, some energy is lost as heat energy. (This is good for warm-blooded creatures like us, because heat energy helps to maintain our body temperature.) In another example, as molecules at a high concentration in one place diffuse and spread out, entropy increases (Figure 2.22).
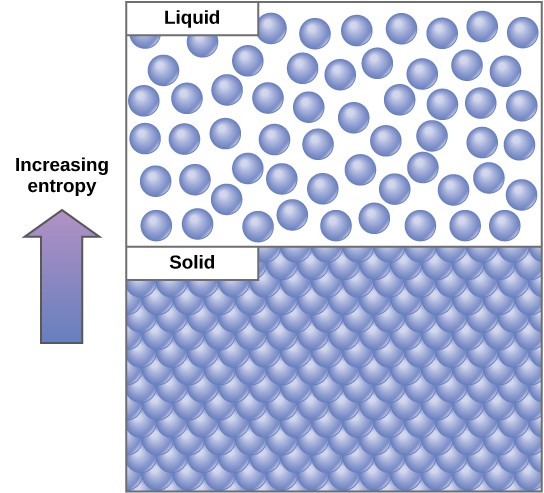
Since living things are highly ordered, they require a constant input of energy. Essentially, living things are in a continuous uphill battle against this constant increase in universal entropy.
2.5 | Carbon
Learning Objectives
By the end of this section, you will be able to:
- Explain why carbon is important for life.
- Describe the role of functional groups in biological molecules.
Cells are made of many complex organic (carbon-containing) molecules, such as proteins and carbohydrates, which are especially important for life. The fundamental component of all of these macromolecules is carbon. Carbon atoms can form covalent bonds with up to four different atoms, making them ideal to form the “backbone” of macromolecules.
2.5.1 Hydrocarbons
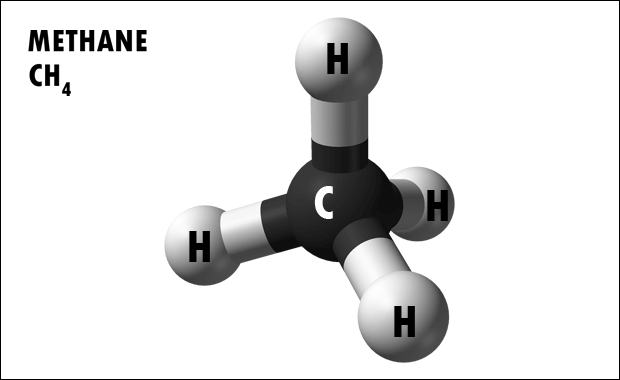
Hydrocarbons are organic molecules consisting entirely of carbon and hydrogen. We often use hydrocarbons as fuels—like the propane in a gas grill or the butane in a lighter. The covalent bonds between the atoms in hydrocarbons store a great amount of energy, which is released when these molecules are burned (oxidized). Methane, an excellent fuel, is the simplest hydrocarbon molecule (Figure 2.23). Hydrocarbons may exist as linear carbon chains, carbon rings, or combinations of both. Furthermore, individual carbon-to- carbon bonds may be single, double, or triple covalent bonds.
2.5.2 Functional Groups
Functional groups are groups of atoms that occur commonly within molecules and confer specific chemical properties to those molecules. They are found attached to the carbon “backbone” of macromolecules. Each of the four types of macromolecules—proteins, lipids, carbohydrates, and nucleic acids—has its own characteristic set of functional groups that contributes greatly to its differing chemical properties and its function in living organisms.
Some of the important functional groups in biological molecules are shown in Figure 2.24. Functional groups are often classified as polar or non-polar, since that determines whether they are hydrophobic or hydrophilic. For example, non-polar methyl groups are hydrophobic and polar hydroxyl groups are hydrophilic. Functional groups can also be classified as acidic or basic, depending on whether they release or accept H+ in solution. Releasing H+ results in a negatively charged functional group, such as phosphate groups or carboxyl groups. Accepting H+ results in a positively charged functional group, such as amino groups.
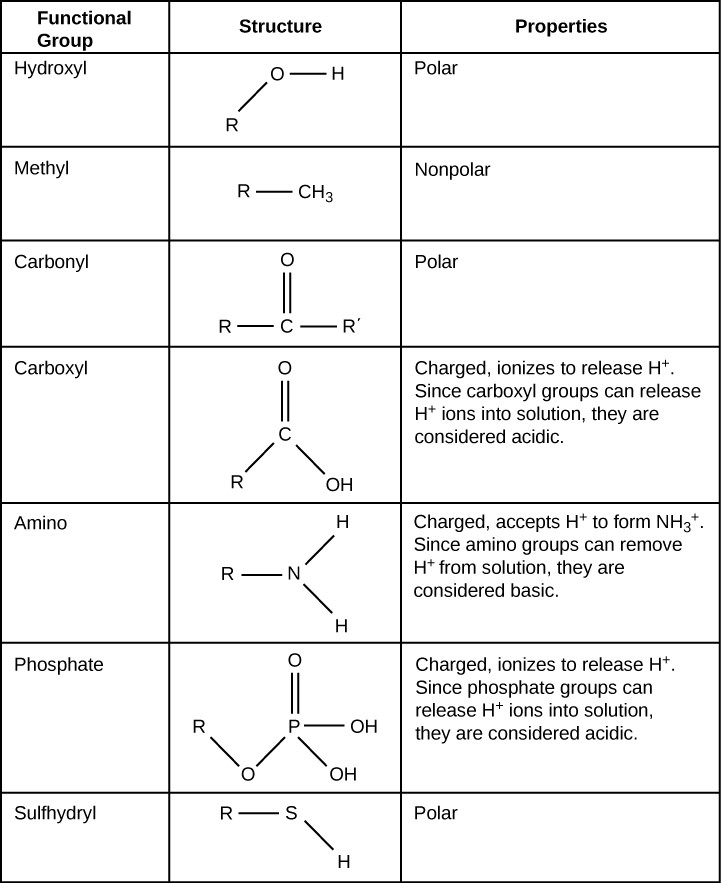