Chapter 11. Cellular Respiration
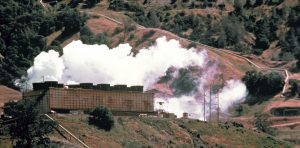
Chapter Outline
- 11.1 Overview of Cellular Respiration
- 11.2 Glycolysis
- 11.3 Pyruvate Oxidation and the Citric Acid Cycle
- 11.4 Oxidative Phosphorylation
- 11.5 Metabolism Without Oxygen
- 11.6 Metabolism of Other Nutrients
- 11.7 Regulation of Cellular Respiration
Introduction
The electrical energy plant in Figure 11.1 converts energy from one form to another form that can be more easily used. This type of generating plant starts with underground thermal energy (heat) and transforms it into electrical energy that will be transported to homes and factories. Like a generating plant, plants and animals must also take in energy from the environment and convert it into a form that their cells can use.
Energy enters an organism’s body in one form and is converted into another form. In plants and other photosynthetic producers, the process of photosynthesis takes in light energy and converts it into chemical energy in the form of glucose. This energy is stored in the chemical bonds of the glucose. Next, a series of metabolic pathways, called cellular respiration, extracts the energy from the bonds in glucose and converts it into a form that all living things can use: ATP.
11.1 | Overview of Cellular Respiration
Learning Objectives
By the end of this section, you will be able to:
- Discuss how electron movement and energy are related in cellular respiration.
- Discuss how breathing is related to cellular respiration.
- Name the four phases of cellular respiration.
As discussed in the previous chapter, oxidation of (removing electrons from) energy-storing molecules like glucose releases energy that can be used to do cellular work. Cellular respirations involves a series of electron transfers from a high energy state in glucose to a low energy state, as part of water. The energy released in this process is used to make ATP, both through substrate-level phosphorylation and oxidative phosphorylation. As electrons are progressively removed from the glucose carbons, glucose is ultimately broken down into 6 molecules of CO2.
The full equation for cellular respiration is listed below.
C6H12O6 + 6 O2 → 6 CO2 + 6 H2O + energy
As you can see, oxygen is required for cellular respiration. Without oxygen to act as the final electron acceptor, glucose cannot be fully broken down to CO2. We breathe air and extract oxygen from it in order to break down glucose (and other nutrients) and produce ATP. When we exhale, we release the CO2 that is the byproduct of glucose breakdown.
Cellular respiration occurs in four phases, that will be discussed in detail below.
Phase 1: Glycolysis
Phase 2: Pyruvate oxidation
Phase 3: The citric acid cycle
Phase 4: Oxidative phosphorylation
11.2 | Glycolysis
Learning Objectives
By the end of this section, you will be able to:
- Describe the overall result in terms of molecules produced in the breakdown of glucose by glycolysis.
- State the output of glycolysis in terms of ATP molecules and NADH molecules produced.
You have read that nearly all of the energy used by living cells resides in the bonds of the monosaccharide glucose. Glycolysis is the first step in the breakdown of glucose to extract energy for cellular metabolism. Nearly all living organisms carry out glycolysis as part of their metabolism. The process does not use oxygen and is therefore anaerobic. Glycolysis takes place in the cytoplasm of both prokaryotic and eukaryotic cells.
Before glycolysis can begin, glucose must be present in the cell. Glucose may be obtained in many ways, including facilitated diffusion across the plasma membrane through carrier proteins, secondary active transport using sodium ion gradients set up by the sodium-potassium pump, breakdown of disaccharides, such as sucrose, to form glucose and other monosaccharides, and breakdown of storage polymers, such as glycogen or starch, to yield glucose.
11.2.1 Simplified Overview of Glycolysis
Glycolysis begins with a single six-carbon glucose molecule and ends with two molecules of a three-carbon sugar called pyruvate. Glycolysis consists of three stages: investment, cleavage, and harvest (Figure 11.2).
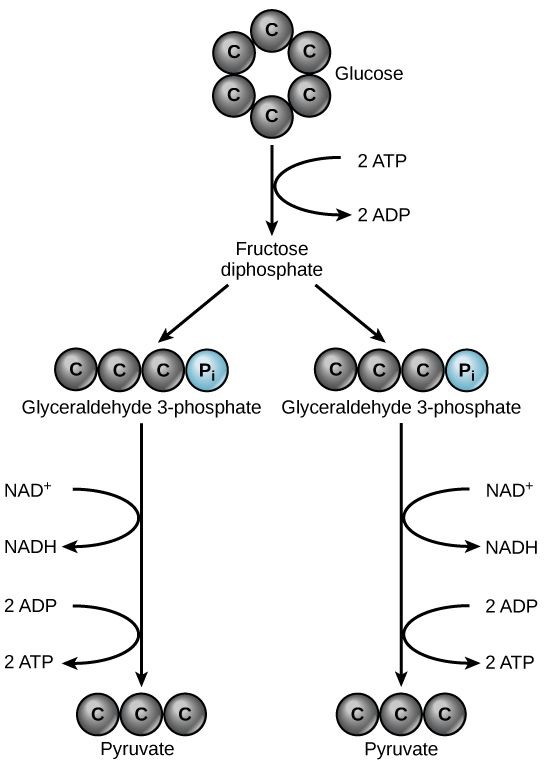
During investment, two molecules of ATP phosphorylate the six-carbon sugar. During cleavage, the six-carbon molecule is split into two three-carbon molecules. During harvest, energy is captured from the two three carbon molecules and stored in the form of ATP and NADH.
11.2.2 Details of Glycolysis
Glycolysis is a ten-step metabolic pathway. A more detailed look at the steps of glycolysis is shown below.
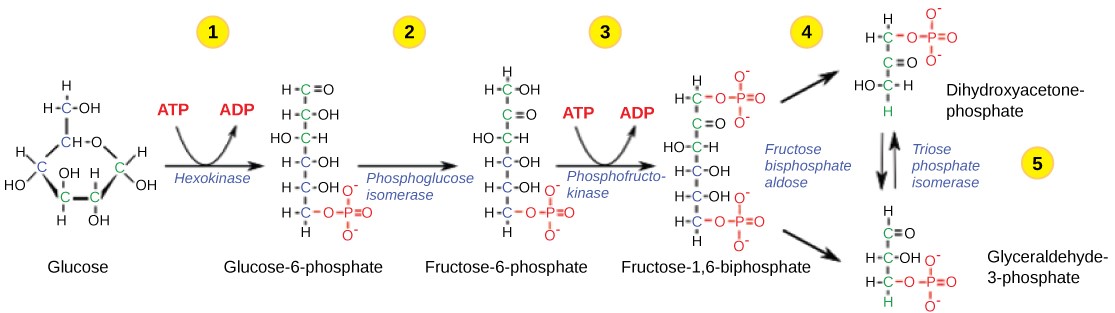
Table 11.1 Steps in the first half of glycolysis.
Step 1 | Investment #1 | The enzyme hexokinase catalyzes the phosphorylation of glucose, using ATP as the source of phosphate, producing glucose-6-phosphate. This reaction prevents the phosphorylated glucose molecule from leaving the cell because it can no longer cross the phospholipid bilayer of the plasma membrane through any transport protein. |
Step 2 | Isomerize | The enzyme phosphogluco-isomerase converts glucose-6-phosphate into one of its isomers, fructose-6-phosphate. (Remember that isomers have the same chemical formula but have different chemical structures.) |
Step 3 | Investment #2 | The enzyme phosphofructokinase catalyzes the phosphorylation of fructose-6-phosphate, using a second ATP, producing fructose-1,6-bisphosphate. |
Step 4 | Cleavage | The enzyme aldolase cleaves 1,6-bisphosphate into two three-carbon isomers: dihydroxyacetone-phosphate (DHAP) and glyceraldehyde-3-phosphate (3PGA) |
Step 5 | Isomerize | The enzyme triose-phosphate isomerase transforms DHAP into its isomer, 3PGA. Thus, the pathway will continue with two molecules of 3PGA. |
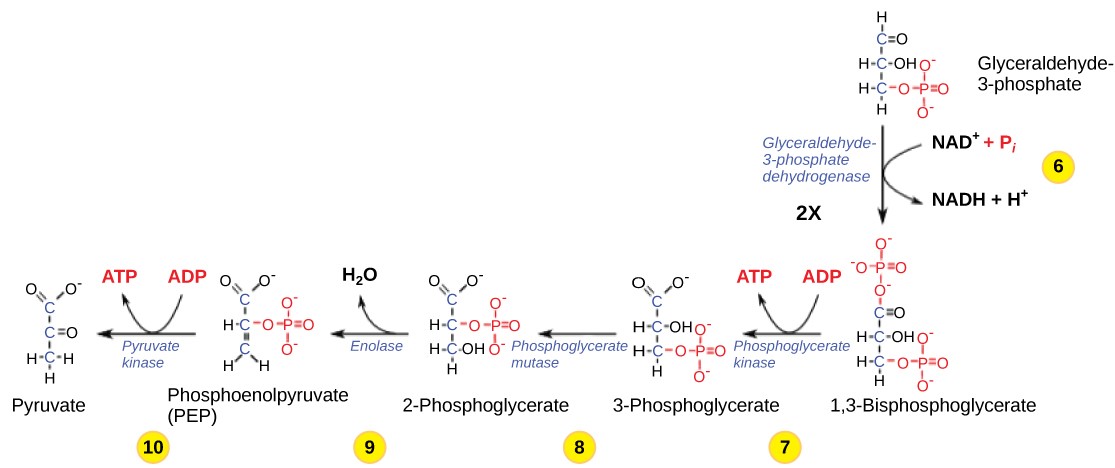
Table 11.2 Steps in the second half of glycolysis.
Step 6 | Produce NADH | The enzyme glyceraldehyde-3-phosphate dehydrogenase catalyzes the oxidation of glyceraldehyde-3-phosphate by transferring high-energy electrons to NAD+ to produce NADH. The sugar is then phosphorylated by the addition of a second phosphate group, producing 1,3-bisphosphoglycerate. Note that the second phosphate group does not require another ATP molecule. |
Step 7 | Produce ATP | The enzyme phosphoglycerate kinase catalyzes the transfer of a high-energy phosphate from 1,3-bisphosphoglycerate to ADP, forming one molecule of ATP. This is an example of substrate-level phosphorylation. The aldehyde group on the 1,3-bisphosphoglycerate is oxidized to a carboxyl group, and 3phosphoglycerate is formed. |
Step 8 | Isomerize | A mutase enzyme moves the remaining phosphate group in 3-phosphoglycerate from the third carbon to the second carbon, producing 2-phosphoglycerate (an isomer of 3-phosphoglycerate). |
Step 9 | Lose water | The enzyme enolase causes 2-phosphoglycerate to lose water (a dehydration reaction), resulting in the formation of a double bond that increases the potential energy in the remaining phosphate bond and produces phosphoenolpyruvate (PEP). |
Step 10 | Produce ATP | The enzyme pyruvate kinase causes the production of a second ATP molecule by substrate-level phosphorylation and the compound pyruvic acid (or its ionized form, pyruvate). |
11.2.3 Outcomes of Glycolysis
Glycolysis starts with glucose and ends with two pyruvate molecules, a total of four ATP molecules and two molecules of NADH. Since two ATP molecules were invested in the first stage of the pathway, the cell has a net gain of 2 ATP molecules and 2 NADH molecules. If the cell cannot catabolize the pyruvate molecules further, it will harvest only two ATP molecules from one molecule of glucose.
QUICK REVIEW OF GLYCOLYSIS:
-
- Splits a six-carbon glucose molecule into 2 three-carbon molecules of pyruvate, using 10 enzyme-catalyzed reactions
- Yields a net gain of 2 ATP and 2 NADH
- Takes place in the cytoplasm
- Can occur without oxygen; is an anaerobic process
11.3 | Pyruvate Oxidation and the Citric Acid Cycle
Learning Objectives
Type your learning objectives here.
- Describe the overall result in terms of molecules produced in the breakdown of pyruvate by pyruvate oxidation and the citric acid cycle.
- State the output of pyruvate oxidation and the citric acid cycle in terms of ATP molecules and NADH molecules produced.
11.3.1 Pyruvate Oxidation
If oxygen is available, aerobic respiration will proceed. In eukaryotic cells, the pyruvate molecules produced at the end of glycolysis are transported into the mitochondria. Once inside the mitochondria, one CO2 will be removed from pyruvate, leaving an acetyl group that will be picked up and activated by a carrier compound called coenzyme A (CoA). The resulting compound is called acetyl CoA. CoA is made from vitamin B5 (pantothenic acid). During the formation of acetyl CoA from pyruvate, 2 electrons are also transferred to NAD+, forming NADH. Acetyl CoA can be used in a variety of ways by the cell, but its major function is to deliver the acetyl group derived from pyruvate to the citric acid cycle, which is the next pathway in glucose catabolism. (Figure 11.5). Since there are two pyruvate molecules formed from each glucose molecule, this step occurs twice. Therefore, these are the first two of the six carbons from the original glucose molecule to be removed as CO2.
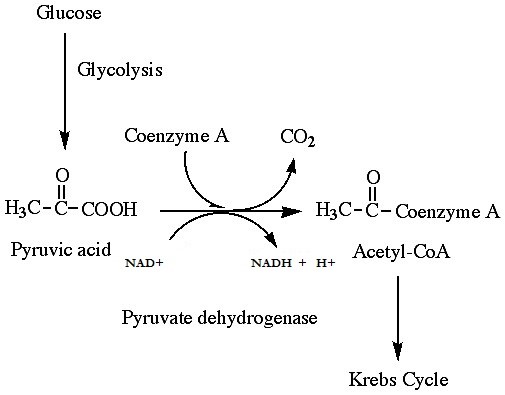
QUICK REVIEW OF PYRUVATE OXIDATION
-
- One carbon atom per pyruvate molecule is released as CO2, or two total CO2 molecules per glucose. 2 acetyl CoA molecules per glucose remain.
- Yields a net gain of 2 NADH per glucose
- Takes place in the mitochondrial matrix
- Only occurs in the presence of oxygen; is an aerobic process
11.3.2 The Citric Acid Cycle
In the presence of oxygen, acetyl CoA delivers its acetyl group to a four-carbon molecule called oxaloacetate, to form a six-carbon molecule called citric acid. The next pathway will harvest the remainder of the extractable energy from what began as a glucose molecule. This single pathway is called the citric acid cycle (also called the TCA cycle or the Krebs cycle).
Like pyruvate oxidation, the citric acid cycle takes place in the matrix of mitochondria. Unlike glycolysis, the citric acid cycle is a closed loop; the last part of the pathway regenerates the compound used in the first step. The eight steps of the cycle are a series of redox, dehydration, hydration, and decarboxylation reactions that produce two carbon dioxide molecules from each two-carbon acetyl group, one GTP directly (which then forms ATP), and reduced forms of NADH and FADH2 (Figure 11.6). This is considered an aerobic pathway because the NADH and FADH2 produced must transfer their electrons to the next pathway in the system, which will use oxygen. If this transfer does not occur, the oxidation steps of the citric acid cycle also do not occur. Note that the citric acid cycle produces very little ATP directly and does not directly consume oxygen.
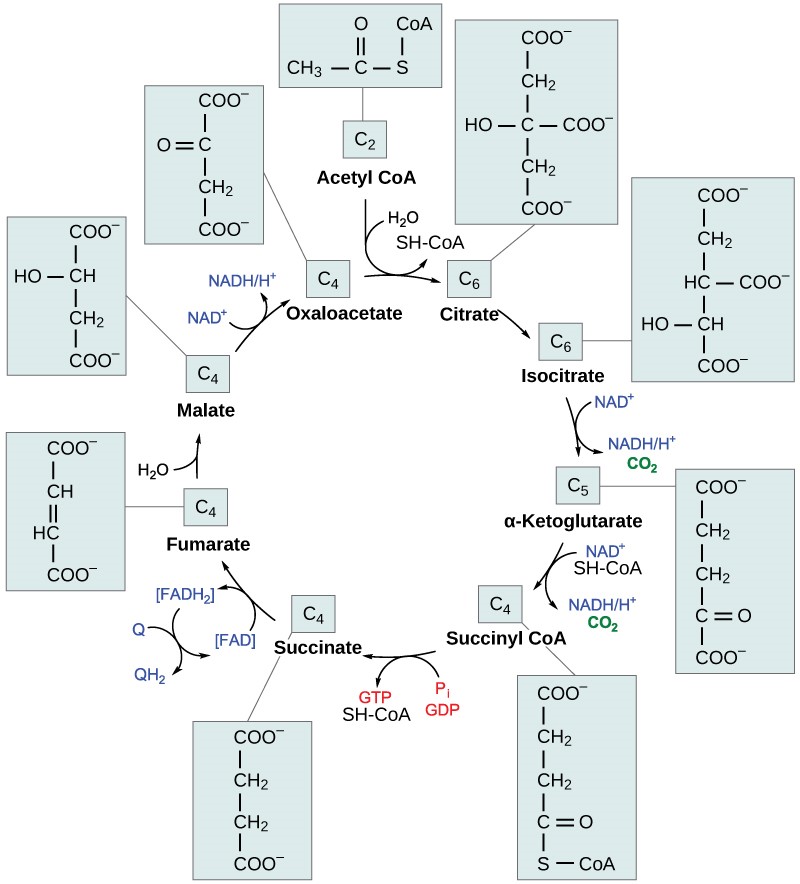
Table 11.3 Steps of the citric acid cycle.
Step 1 | Formation of citric acid (Top of Figure 11.6) | A two-carbon acetyl group is combined with a four-carbon oxaloacetate to form a six-carbon citric acid. This step is irreversible because it is highly exergonic. |
Step 2 | Isomerization | Citric acid is isomerized to isocitrate. |
Step 3 | CO2 produced | Isocitrate is oxidized, producing a five-carbon α-ketoglutarate and CO2. NAD+ is reduced to NADH. |
Step 4 | CO2 produced | α-ketoglutarate and CoA form succinyl-CoA and CO2. NAD+ is reduced to NADH. The bond between the succinate group and CoA hold a great deal of energy that will later be used to produce GTP or ATP in the next step. |
Step 5 | ATP or GTP produced | The bond between succinate and CoA is broken, which provides energy for the substrate-level phosphorylation of GDP to form GTP, which is quickly converted to ATP. Plants and bacteria form ATP instead. This is the only substrate-level phosphorylation in the citric acid cycle. |
Step 6 | FADH2 produced | Succinate is converted to fumarate; two hydrogen atoms are transferred to FAD, producing FADH2. |
Step 7 | Hydration | Fumarate is converted to malate. |
Step 8 | Oxaloacetate is regenerated | Malate is oxidized to form oxaloacetate and NAD+ is reduced to NADH. Since the end product re-creates one of the starting products, this pathway is a cycle. |
By the end of the citric acid cycle, all six carbon atoms from the original glucose molecule have been released as CO2. Each turn of the cycle forms three NADH molecules and one FADH2 molecule. These carriers will be used in the last pathway of aerobic respiration to produce ATP. One GTP or ATP is also made by substrate-level phosphorylation in each cycle. Since the original glucose molecule produces two acetyl CoA, two turns of the cycle occur for each glucose molecule.
QUICK REVIEW OF THE CITRIC ACID CYCLE
-
- The cycle begins with the two acetyl-CoA from pyruvate oxidation. By the end of the cycle, all of these carbons are released as CO2.
- Two GTP or ATP per glucose are made by substrate-level phosphorylation
- Six NAD+ are reduced to NADH and two FAD are reduced to FADH2 per glucose
- Takes place in the mitochondrial matrix
- Only occurs in the presence of oxygen; is an aerobic process
11.4 | Oxidative Phosphorylation
Learning Objectives
By the end of this section, you will be able to:
- Describe how electrons move through the electron transport chain.
- Explain how a proton (H+) gradient is established and maintained by the electron transport chain.
- Explain how the electron transport chain and chemiosmosis together comprise oxidative phosphorylation.
You have just read about three pathways in glucose catabolism – glycolysis, pyruvate oxidation, and the citric acid cycle. Two of these pathways generate ATP a small amount of ATP by substrate-level phosphorylation. However, most of the ATP generated during the aerobic catabolism of glucose is not generated directly from these pathways. Rather, it is derived during a process called oxidative phosphorylation, which is made up of two parts: electron transport and chemiosmosis.
11.4.1 The Electron Transport Chain
The electron transport chain (ETC) is a series of proteins embedded into the inner membrane of mitochondria and the plasma membrane of prokaryotes. The ETC consists of four protein complexes (labeled I through IV in Figure 11.7) and two mobile accessory electron carriers. Electron transport is a series of redox reactions that resemble a relay race or bucket brigade in that electrons are passed rapidly from one component to the next.
The NADH and FADH2 that were produced during the previous phases of glucose catabolism provide the electrons to fuel the ETC. As these electrons are transported down the ETC, the electrons move from higher to lower energy states, and exergonic process. The energy released is used to shuttle hydrogen ions (H+) from the mitochondrial matrix, across the inner membrane, into the intermembrane space. This produces an H+ gradient, and endergonic process. The energy stored in the electrochemical gradient is then used to make ATP during chemiosmosis.
It is important to note that electrons from NADH enter the ETC at Complex I and then proceed to Complexes III and IV. However, electrons from FADH2 enter the ETC at Complex II before proceeding to Complexes III and IV (Figure 11.8). Overall, NADH contains sufficient energy to transport three hydrogen ions into the intermembrane space, and FADH2 contains sufficient energy to transport two hydrogen ions into the intermembrane space. In this way, the hydrogen ion gradient is established and maintained between the two compartments separated by the inner mitochondrial membrane.
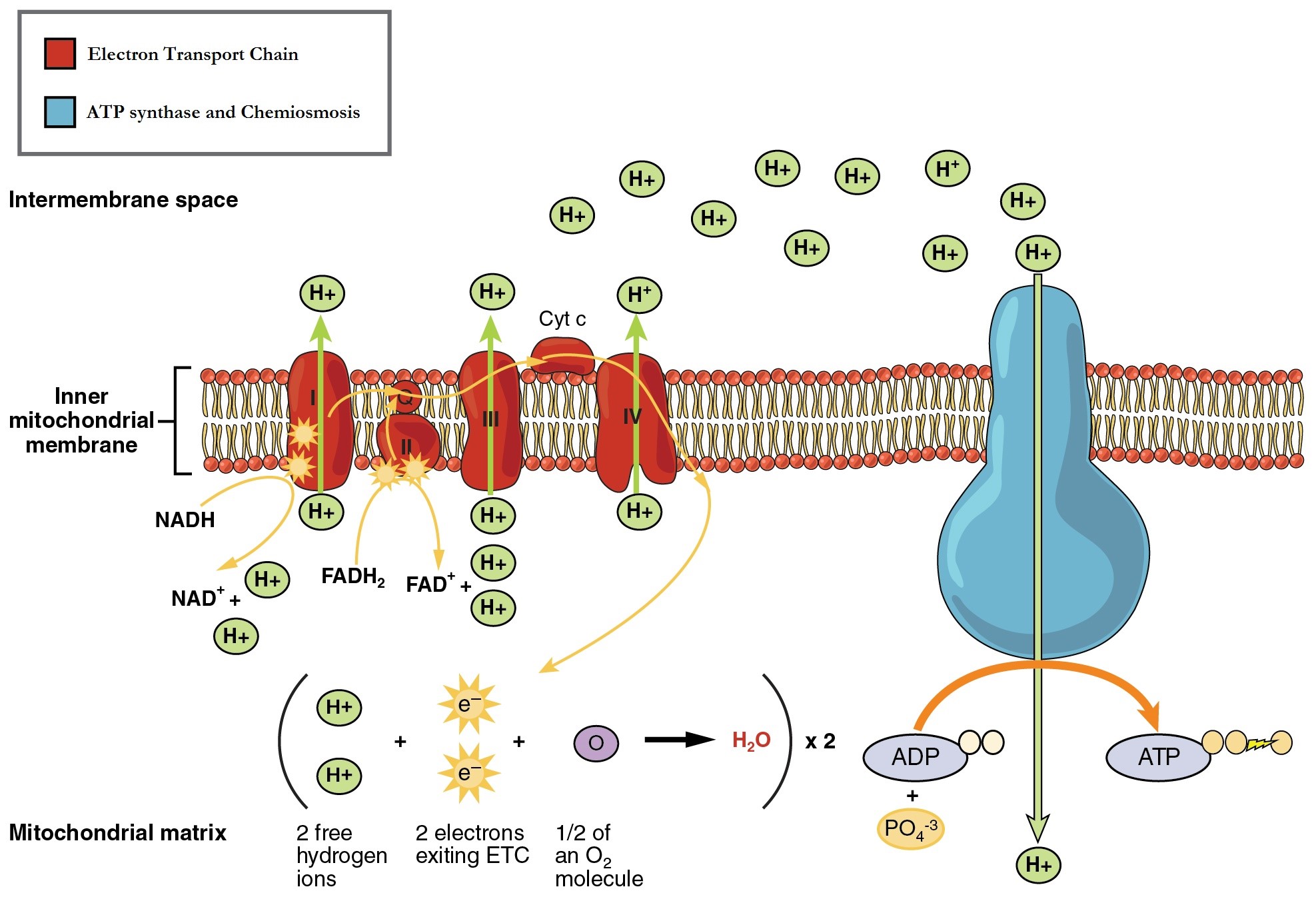
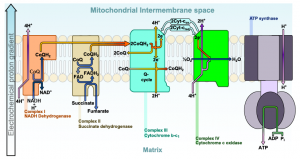
Table 11.4 The movement of electrons through the mitochondrial electron transport chain.
Complex I | Integral membrane protein complex | Complex I is composed of flavin mononucleotide (FMN) and an iron-sulfur (Fe-S)-containing protein. The enzyme in complex I is NADH dehydrogenase. Two electrons are transferred from NADH to Complex I, providing the energy for it to transport a hydrogen ion from the matrix into the intermembrane space. Electrons that enter the ETC at Complex I bypass Complex II and are transferred to Coenzyme Q. |
Complex II | Integral membrane protein complex | Complex II directly receives electrons from the oxidation of FADH2 to FAD, using the enzyme succinate dehydrogenase (from the citric acid cycle) and does not pass through complex I. This enzyme and FADH2 form a small complex that delivers electrons directly to the electron transport chain, bypassing the first complex. Since these electrons bypass and thus do not energize the proton pump in the first complex, fewer ATP molecules are made from the FADH2 electrons. |
Coenzyme Q | Coenzyme embedded in the inner mitochondria membrane | Ubiquinone (coenzyme Q) receives electrons from complex I and complex II and delivers them to Complex III. |
Complex III | Integral membrane protein complex | Complex III is composed of cytochrome b and c proteins (this complex is also called cytochrome oxidoreductase). Cytochrome proteins have a heme group, which is similar to the heme in hemoglobin, except that it carries electrons rather than oxygen. Complex III uses the energy of the electrons obtained from Q to transport another hydrogen ion from the matrix to the intermembrane space. |
Cytochrome c | Small protein associated with the outside of the inner mitochondrial membrane | The electrons are then transported to cytochrome c, which transfers them to Complex IV. |
Complex IV | Integral membrane protein complex | Complex IV is composed of cytochrome proteins c, a, and a3. This complex contains two heme groups and three copper ions. Complex IV uses the energy of the electrons obtained from complex III to pump another hydrogen ion to the intermembrane space. Complex IV also aids in the splitting of molecular oxygen (O2), so that it picks up two electrons (plus two hydrogen ions from the surrounding medium) to make water. The removal of the hydrogen ions from the system contributes to the ion gradient used in the process of chemiosmosis. |
11.4.2 Chemiosmosis
As the ETC works, the concentration gradient of H+ ions across the membrane establishes an electrochemical gradient. The hydrogen ions’ positive charge comprises the electrical part of the gradient, and their aggregation on one side of the membrane comprises the chemical part of the gradient. Driven by their electrochemical gradient, the hydrogen ions tend to move back across the inner membrane into the matrix. They pass through an integral membrane protein called ATP synthase (Figure 11.9). This complex protein acts as a tiny turbine, spun by the force of the hydrogen ions diffusing through it. The turning of this molecular machine creates mechanical energy, which facilitates the addition of a phosphate to ADP, forming ATP.
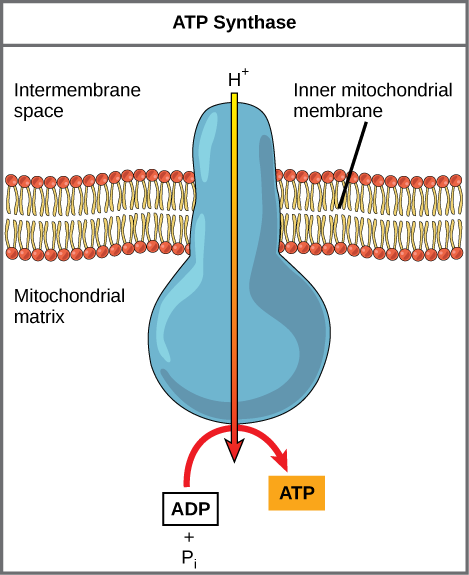
Chemiosmosis (Figure 11.7) is used to generate 90% of the ATP made during aerobic glucose catabolism. The overall result of these reactions is the production of ATP from the energy of the electrons that were originally part of a glucose molecule.
Recall that the production of ATP using the process of chemiosmosis in mitochondria is called oxidative phosphorylation. The ETC is the only part of glucose metabolism that directly uses atmospheric oxygen. Oxygen continuously diffuses into plant and bacterial cells; in animals, it enters the body through the respiratory and circulatory systems, from which it diffuses into cells. In mitochondria, oxygen gas is the terminal electron acceptor for the ETC. If oxygen is not present, the ETC becomes backed up and eventually shuts down. With no ETC to accept electrons from NADH and FADH2, these electron carriers remain reduced and are not available to accept additional electrons during pyruvate oxidation and the citric acid cycle. Therefore, these pathways also shut down. Most cells die in the absence of oxygen for this reason.
Concept Check
Dinitrophenol (DNP) is an uncoupler that makes the inner mitochondrial membrane leaky to protons. It was used until 1938 as a weight-loss drug. What effect would you expect DNP to have on the change in pH across the inner mitochondrial membrane? Why do you think this might be an effective weight-loss drug? DNP was removed from the FDA’s approved list in 1938 after people died from high fevers. Why do you think DNP would cause people to overheat?
Concept Check
The poison cyanide inhibits cytochrome c oxidase, a component of the ETC. If cyanide poisoning occurs, would you expect the pH of the intermembrane space to increase or decrease? What effect would cyanide have on ATP synthesis?
11.4.3 ATP Yield
The number of ATP molecules generated from the catabolism of glucose varies. For example, the number of hydrogen ions that the electron transport chain complexes can pump through the membrane varies between species. Overall, in living systems, these pathways of glucose catabolism extract about 34% of the energy gained in glucose. The maximum yield of ATP by oxidative phosphorylation under ideal conditions is approximately 34 ATP per glucose molecule. Since four ATP are made by substrate-level phosphorylation, the total maximum yield is 38 ATP per glucose molecule.
11.5 | Metabolism without Oxygen
Learning Objectives
By the end of this section, you will be able to:
- Discuss why fermentation is necessary in the absence of oxygen.
- Compare the ATP yield of aerobic and anaerobic metabolism.
- Describe the type of fermentation that readily occurs in animal cells and the conditions that initiate that fermentation.
In aerobic respiration, ATP is produced by the electron transport chain using the energy of high-energy electrons carried by NADH or FADH2, and the final electron acceptor is an oxygen molecule. However, in some organisms and under some conditions, ATP can be produced in the absence of oxygen.
11.5.1 Anaerobic Cellular Respiration
In some organisms, molecules other than oxygen are used as the final electron acceptor. If an inorganic molecule is used as the final electron acceptor, the process is called anaerobic cellular respiration. Certain prokaryotes use anaerobic respiration to produce ATP. For example, the group of Archaea called methanogens uses carbon dioxide to oxidize NADH, producing methane. These microorganisms are found in soil and in the digestive tracts of ruminants, such as cows and sheep. Similarly, sulfur bacteria use sulfate to regenerate NAD+ from NADH, producing hydrogen sulfide (Figure 11.10).
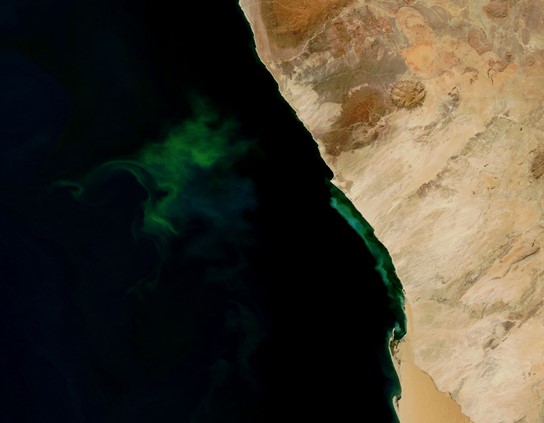
11.5.2 Lactic Acid Fermentation
If neither aerobic nor anaerobic respiration occur, NADH accumulates in cells. If a cell does not have oxidized electron carriers, it cannot break glucose down because there is nowhere to put the electrons. In other words, NADH must be re-oxidized to NAD+ for reuse as an electron carrier for glycolysis to continue. Some living systems use an organic molecule as the final electron acceptor, in a process referred to as fermentation.
The fermentation method used by animals and certain bacteria, like those that make yogurt, is lactic acid fermentation (Figure 11.11). This type of fermentation is used routinely in mammalian red blood cells and in skeletal muscle cells that have an insufficient oxygen supply to allow aerobic respiration to continue (that is, in muscles used to the point of fatigue). The enzyme used in this reaction is lactate dehydrogenase (LDH). The reaction can proceed in either direction, but the reaction from left to right is inhibited by acidic conditions. Lactic acid accumulation was once believed to cause muscle stiffness, fatigue, and soreness, although more-recent research disputes this hypothesis. In muscles, lactic acid accumulation must be removed by the blood circulation and the lactate brought to the liver for further metabolism. Once the lactic acid has been removed from the muscle and circulated to the liver, it can be reconverted into pyruvic acid and further catabolized for energy.
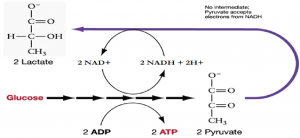
11.5.3 Alcohol Fermentation
Another familiar fermentation process is the two-step process of alcohol fermentation that produces the alcohol ethanol (Figure 11.12). The first step releases CO2 gas and acetaldehyde. The second step oxidizes NADH to NAD+ and reduces acetaldehyde to ethanol.
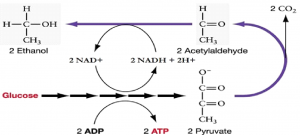
The first reaction is catalyzed by pyruvate decarboxylase with a coenzyme derived from vitamin B1 (thiamine). A carboxyl group is removed from pyruvate, releasing CO2. The loss of carbon dioxide reduces the size of the molecule by one carbon, making acetaldehyde. The second reaction is catalyzed by alcohol dehydrogenase to oxidize NADH to NAD+ and reduce acetaldehyde to ethanol. The fermentation of pyruvic acid by yeast produces the ethanol found in alcoholic beverages. Ethanol is toxic to yeast, limiting the alcohol levels of fermented beverages. The tolerance of yeast is variable, ranging from about 5 percent to 21 percent, depending on the yeast strain and environmental conditions.
11.5.4 Other Types of Fermentation
Other fermentation methods occur in some types of bacteria. Many prokaryotes are “facultatively” anaerobic, meaning that they can switch between aerobic respiration and fermentation, depending on the availability of oxygen. Certain prokaryotes, like Clostridia, are obligate anaerobes, which live and grow in the absence of molecular oxygen. Oxygen is poisonous to these microorganisms and kills them on exposure. It should be noted that all forms of fermentation, except lactic acid fermentation, produce gas. The production of particular types of gas is used as an indicator of the fermentation of specific carbohydrates, which plays a role in the laboratory identification of the bacteria.
Regardless of the type of fermentation, the purpose is always the same: to ensure an adequate supply of NAD+ so that glycolysis can continue and ATP can be harvested from the breakdown of glucose. Note that fermentation does not capture any energy; in fact, it wastes energy by returning the electrons held by NADH to pyruvate. The only energy produced in fermenting cells is the two ATP created during glycolysis. However, without fermentation to produce NAD+, glycolysis could not continue and the cell would die.